Research.Pdf (4.771Mb)
Total Page:16
File Type:pdf, Size:1020Kb
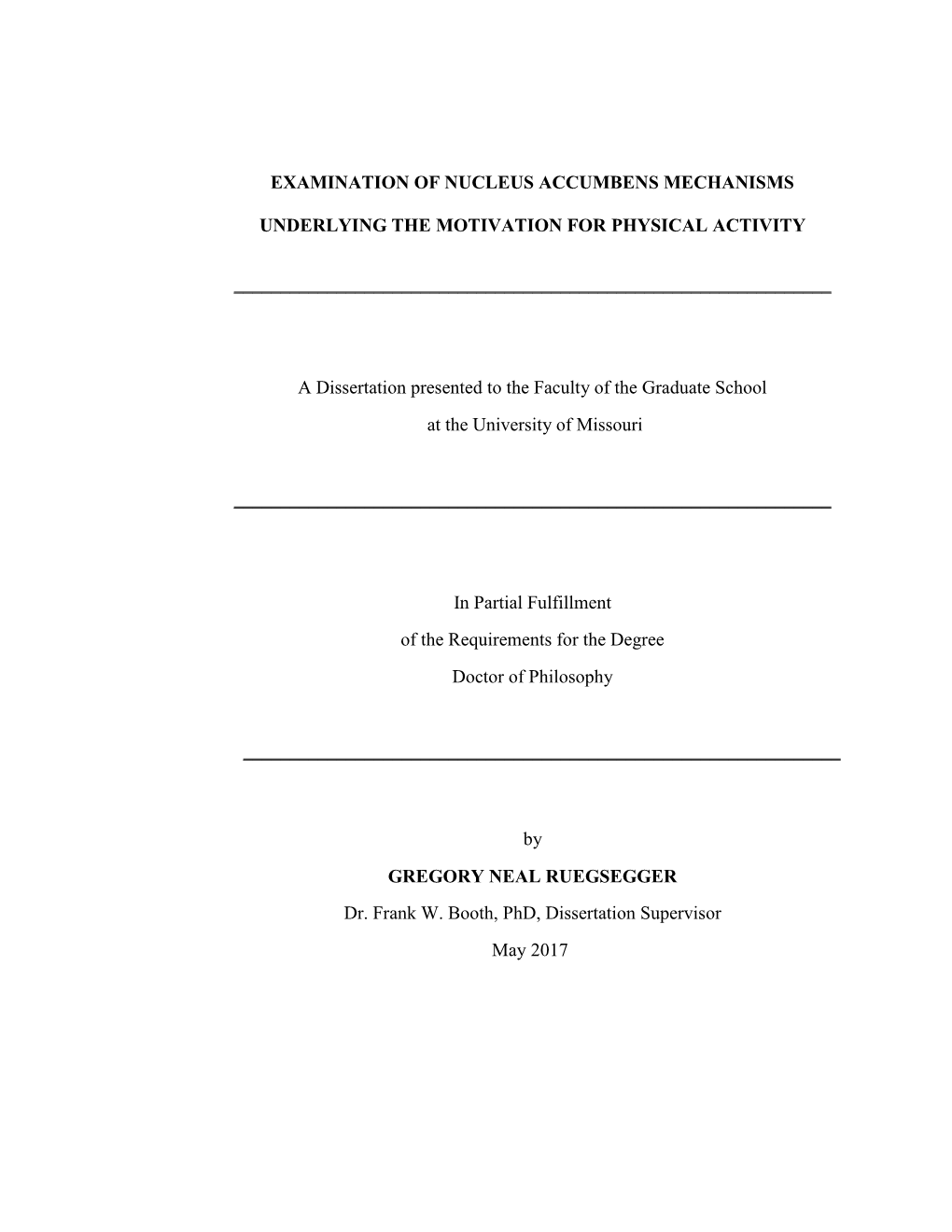
Load more
Recommended publications
-
Genome-Wide Screen of Otosclerosis in Population Biobanks
medRxiv preprint doi: https://doi.org/10.1101/2020.11.15.20227868; this version posted November 16, 2020. The copyright holder for this preprint (which was not certified by peer review) is the author/funder, who has granted medRxiv a license to display the preprint in perpetuity. It is made available under a CC-BY-NC-ND 4.0 International license . 1 Genome-wide Screen of Otosclerosis in 2 Population Biobanks: 18 Loci and Shared 3 Heritability with Skeletal Structure 4 Joel T. Rämö1, Tuomo Kiiskinen1, Juha Karjalainen1,2,3,4, Kristi Krebs5, Mitja Kurki1,2,3,4, Aki S. 5 Havulinna6, Eija Hämäläinen1, Paavo Häppölä1, Heidi Hautakangas1, FinnGen, Konrad J. 6 Karczewski1,2,3,4, Masahiro Kanai1,2,3,4, Reedik Mägi5, Priit Palta1,5, Tõnu Esko5, Andres Metspalu5, 7 Matti Pirinen1,7,8, Samuli Ripatti1,2,7, Lili Milani5, Antti Mäkitie9, Mark J. Daly1,2,3,4,10, and Aarno 8 Palotie1,2,3,4 9 1. Institute for Molecular Medicine Finland (FIMM), Helsinki Institute of Life Science (HiLIFE), University of 10 Helsinki, Helsinki, Finland 11 2. Program in Medical and Population Genetics, Broad Institute of Harvard and MIT, Cambridge, 12 Massachusetts, USA 13 3. Stanley Center for Psychiatric Research, Broad Institute of Harvard and MIT, Cambridge, Massachusetts, 14 USA 15 4. Analytic and Translational Genetics Unit, Massachusetts General Hospital, Boston, Massachusetts, USA 16 5. Estonian Genome Center, University of Tartu, Tartu, Estonia, Institute of Molecular and Cell Biology, 17 University of Tartu, Tartu, Estonia 18 6. Finnish Institute for Health and Welfare, Helsinki, Finland 19 7. Department of Public Health, Clinicum, Faculty of Medicine, University of Helsinki, Helsinki, Finland 20 8. -
Mutational Mechanisms That Activate Wnt Signaling and Predict Outcomes in Colorectal Cancer Patients William Hankey1, Michael A
Published OnlineFirst December 6, 2017; DOI: 10.1158/0008-5472.CAN-17-1357 Cancer Genome and Epigenome Research Mutational Mechanisms That Activate Wnt Signaling and Predict Outcomes in Colorectal Cancer Patients William Hankey1, Michael A. McIlhatton1, Kenechi Ebede2, Brian Kennedy3, Baris Hancioglu3, Jie Zhang4, Guy N. Brock3, Kun Huang4, and Joanna Groden1 Abstract APC biallelic loss-of-function mutations are the most prevalent also exhibiting unique changes in pathways related to prolifera- genetic changes in colorectal tumors, but it is unknown whether tion, cytoskeletal organization, and apoptosis. Apc-mutant ade- these mutations phenocopy gain-of-function mutations in the nomas were characterized by increased expression of the glial CTNNB1 gene encoding b-catenin that also activate canonical nexin Serpine2, the human ortholog, which was increased in WNT signaling. Here we demonstrate that these two mutational advanced human colorectal tumors. Our results support the mechanisms are not equivalent. Furthermore, we show how hypothesis that APC-mutant colorectal tumors are transcription- differences in gene expression produced by these different ally distinct from APC-wild-type colorectal tumors with canonical mechanisms can stratify outcomes in more advanced human WNT signaling activated by other mechanisms, with possible colorectal cancers. Gene expression profiling in Apc-mutant and implications for stratification and prognosis. Ctnnb1-mutant mouse colon adenomas identified candidate Significance: These findings suggest that colon adenomas genes for subsequent evaluation of human TCGA (The Cancer driven by APC mutations are distinct from those driven by WNT Genome Atlas) data for colorectal cancer outcomes. Transcrip- gain-of-function mutations, with implications for identifying tional patterns exhibited evidence of activated canonical Wnt at-risk patients with advanced disease based on gene expression signaling in both types of adenomas, with Apc-mutant adenomas patterns. -
Engineered Type 1 Regulatory T Cells Designed for Clinical Use Kill Primary
ARTICLE Acute Myeloid Leukemia Engineered type 1 regulatory T cells designed Ferrata Storti Foundation for clinical use kill primary pediatric acute myeloid leukemia cells Brandon Cieniewicz,1* Molly Javier Uyeda,1,2* Ping (Pauline) Chen,1 Ece Canan Sayitoglu,1 Jeffrey Mao-Hwa Liu,1 Grazia Andolfi,3 Katharine Greenthal,1 Alice Bertaina,1,4 Silvia Gregori,3 Rosa Bacchetta,1,4 Norman James Lacayo,1 Alma-Martina Cepika1,4# and Maria Grazia Roncarolo1,2,4# Haematologica 2021 Volume 106(10):2588-2597 1Department of Pediatrics, Division of Stem Cell Transplantation and Regenerative Medicine, Stanford School of Medicine, Stanford, CA, USA; 2Stanford Institute for Stem Cell Biology and Regenerative Medicine, Stanford School of Medicine, Stanford, CA, USA; 3San Raffaele Telethon Institute for Gene Therapy, Milan, Italy and 4Center for Definitive and Curative Medicine, Stanford School of Medicine, Stanford, CA, USA *BC and MJU contributed equally as co-first authors #AMC and MGR contributed equally as co-senior authors ABSTRACT ype 1 regulatory (Tr1) T cells induced by enforced expression of interleukin-10 (LV-10) are being developed as a novel treatment for Tchemotherapy-resistant myeloid leukemias. In vivo, LV-10 cells do not cause graft-versus-host disease while mediating graft-versus-leukemia effect against adult acute myeloid leukemia (AML). Since pediatric AML (pAML) and adult AML are different on a genetic and epigenetic level, we investigate herein whether LV-10 cells also efficiently kill pAML cells. We show that the majority of primary pAML are killed by LV-10 cells, with different levels of sensitivity to killing. Transcriptionally, pAML sensitive to LV-10 killing expressed a myeloid maturation signature. -
Antibody-Drug Conjugates: Possibilities and Challenges
Review Article Antibody-Drug Conjugates: Possibilities and Challenges Mohammad-Reza Nejadmoghaddam 1,2, Arash Minai-Tehrani 2, Ramin Ghahremanzadeh 2, Morteza Mahmoudi 1, Rassoul Dinarvand 1,3, and Amir-Hassan Zarnani 4,5,6 1. Nanotechnology Research Center, Faculty of Pharmacy, Tehran University of Medical Sciences, Tehran, Iran 2. Nanobiotechnology Research Center, Avicenna Research Institute, ACECR, Tehran, Iran 3. Department of Pharmaceutics, Faculty of Pharmacy, Tehran University of Medical Sciences, Tehran, Iran 4. Department of Immunology, Faculty of Public Health, Tehran University of Medical Sciences, Tehran, Iran 5. Reproductive Immunology Research Center, Avicenna Research Institute, ACECR, Tehran, Iran 6. Immunology Research Center, Iran University of Medical Sciences, IUMS, Tehran, Iran * Corresponding authors: Abstract Rassoul Dinarvand, Pharm D., The design of Antibody Drug Conjugates (ADCs) as efficient targeting agents for tu- PhD., Nanotechnology Research mor cell is still in its infancy for clinical applications. This approach incorporates the Center, Faculty of Pharmacy, Tehran University of Medical antibody specificity and cell killing activity of chemically conjugated cytotoxic agents. Sciences, Tehran, Iran Antibody in ADC structure acts as a targeting agent and a nanoscale carrier to deliv- er a therapeutic dose of cytotoxic cargo into desired tumor cells. Early ADCs encoun- Amir-Hassan Zarnani, D.M.T., Ph.D., Reproductive Immunology tered major obstacles including, low blood residency time, low penetration capacity to Research Center, Avicenna tumor microenvironment, low payload potency, immunogenicity, unusual off-target Research Institute, ACECR, toxicity, drug resistance, and the lack of stable linkage in blood circulation. Although Tehran, Iran extensive studies have been conducted to overcome these issues, the ADCs based Tel: +98 21 64121014, 22432020 Fax: +98 21 66959052, 22432021 therapies are still far from having high-efficient clinical outcomes. -
Allicin Inhibits SARS-Cov-2 Replication and Abrogates The
bioRxiv preprint doi: https://doi.org/10.1101/2021.05.15.444275; this version posted June 24, 2021. The copyright holder for this preprint (which was not certified by peer review) is the author/funder, who has granted bioRxiv a license to display the preprint in perpetuity. It is made available under aCC-BY-ND 4.0 International license. Allicin inhibits SARS-CoV-2 replication and abrogates the antiviral host response in the Calu-3 proteome Authors: Kirstin Mösbauer1,2#, Verena Nadin Fritsch3#, Lorenz Adrian4,5, Jörg Bernhardt6, Martin Clemens Horst Gruhlke7, Alan John Slusarenko7, Daniela Niemeyer1,2 and Haike Antelmann3* Departments & Institutions: 1Institute of Virology, Charité-Universitätsmedizin Berlin, Freie Universität Berlin, and Berlin Institute of Health (BIH), Charitéplatz 1, 10117, Berlin, Germany. 2German Centre for Infection Research (DZIF), Berlin, Germany. 3Freie Universität Berlin, Institute for Biology-Microbiology, D-14195 Berlin, Germany 4Department Environmental Biotechnology, Helmholtz Centre for Environmental Research-UFZ, Leipzig, Germany 5 Fachgebiet Geobiotechnologie, Technische Universität Berlin, Germany 6Institute for Microbiology, University of Greifswald, D-17489 Greifswald, Germany 7Department of Plant Physiology, RWTH Aachen University, 52056 Aachen, Germany Running title: Allicin inhibits replication of SARS-CoV-2 in Vero E6 and Calu-3 cells *Corresponding author: Haike Antelmann, Institute for Biology-Microbiology, Freie Universität Berlin, Königin-Luise-Strasse 12-16, D-14195 Berlin, Germany, Tel: +49-(0)30-838-51221, Fax: +49-(0)30-838-451221 E-mail: [email protected] # Authors contributed equally to this work: Kirstin Mösbauer, Verena Nadin Fritsch Key words: SARS-CoV-2/ allicin/ Vero E6/ Calu-3/ proteome 1 bioRxiv preprint doi: https://doi.org/10.1101/2021.05.15.444275; this version posted June 24, 2021. -
Anti-TPBG / 5T4 Antibody [B3F1] (ARG10851)
Product datasheet [email protected] ARG10851 Package: 100 μg anti-TPBG / 5T4 antibody [B3F1] Store at: -20°C Summary Product Description Mouse Monoclonal antibody [B3F1] recognizes TPBG / 5T4. The mouse 5T4 monoclonal antibody, clone B3F1, defines the human 5T4 oncofoetal antigen, a highly glycosylated protein expressed by trophoblast and a few specialized adult epithelia. Up-regulation of 5T4 expression in some cancers is associated with poor clinical outcome; overexpression of human 5T4 cDNA in epithelial cells can alter their morphology and motility, supporting a role for such functions in cancer and development. Tested Reactivity Ms Tested Application ELISA, FACS, FuncSt, ICC/IF, WB Host Mouse Clonality Monoclonal Clone B3F1 Isotype IgG2a Target Name TPBG / 5T4 Antigen Species Mouse Epitope Distal LRR domain of m5T4 Conjugation Un-conjugated Alternate Names 5T4 oncofetal trophoblast glycoprotein; WAIF1; 5T4; Trophoblast glycoprotein; 5T4AG; 5T4 oncofetal antigen; M6P1; 5T4 oncotrophoblast glycoprotein; Wnt-activated inhibitory factor 1 Application Instructions Application table Application Dilution ELISA Assay-dependent FACS Assay-dependent FuncSt Assay-dependent ICC/IF Assay-dependent WB Assay-dependent Application Note Functional study: This antibody inhibits the chemotactic migration towards CXCL12 exhibited by differentiating WT-ES cells. IC50 for the maximally inhibitory mAb B3F1 was 2.2 µg±0.8. * The dilutions indicate recommended starting dilutions and the optimal dilutions or concentrations should be determined by the scientist. Calculated Mw 46 kDa Properties Form Liquid Purification Purification with Protein A. www.arigobio.com 1/2 Buffer PBS and 0.02% Sodium azide. Preservative 0.02% Sodium azide Storage instruction For continuous use, store undiluted antibody at 2-8°C for up to a week. -
Trophoblast Glycoprotein Promotes Pancreatic Ductal Adenocarcinoma Cell Metastasis Through Wnt/Planar Cell Polarity Signaling
MOLECULAR MEDICINE REPORTS 12: 503-509, 2015 Trophoblast glycoprotein promotes pancreatic ductal adenocarcinoma cell metastasis through Wnt/planar cell polarity signaling PING HE1*, SHUHENG JIANG1,2*, MINGZE MA1, YANG WANG1,2, RONGKUN LI1, FANG FANG1, GUANGANG TIAN1 and ZHIGANG ZHANG1 1State Key Laboratory of Oncogenes and Related Genes, Shanghai Cancer Institute, Renji Hospital, Shanghai Jiao Tong University School of Medicine, Shanghai 200240; 2Cancer Institute, Shanghai Medical College of Fudan University, Shanghai 200032, P.R. China Received May 16, 2014; Accepted February 9, 2015 DOI: 10.3892/mmr.2015.3412 Abstract. Trophoblast glycoprotein (TPBG), a 72 kDa glyco- Introduction protein was identified using a monoclonal antibody, which specifically binds human trophoblast. The expression of Pancreatic ductal adenocarcinoma (PDAC), usually referred to TPBG in normal tissues is limited; however, it is upregulated as pancreatic cancer, is a highly aggressive malignant tumor. It in numerous types of cancer. When TPBG is expressed at a is the fourth leading cause of cancer‑associated mortality with high level, this usually indicates a poor clinical outcome. In an estimated 37,390 fatalities in the USA and 227,000 fatali- the present study, it was demonstrated that TPBG was more ties globally per year (1). Patients with PDAC usually present commonly observed in human pancreatic ductal adenocar- with locally advanced, unresectable or metastatic disease and cinoma (PDAC) compared with normal pancreatic tissue. the majority of patients suffer significant pain (2). Despite the Immunohistochemical analysis of PDAC tissue microarrays developments in the detection and management of PDAC, indicated that the expression of TPBG in PDAC tissues was the five‑year relative survival rate has not changed (3). -
Human Cellexp™ TPBG, Human Recombinant
FOR RESEARCH ONLY! 06/20 Human CellExp™ TPBG, Human Recombinant CATALOG NO: P1553-10 10 µg P1553-50 50 µg ALTERNATE NAMES: Trophoblast glycoprotein, 5T4 oncofetal antigen, 5T4 oncofetal trophoblast glycoprotein, 5T4 oncotrophoblast glycoprotein, M6P1, Wnt-activated inhibitory factor 1, WAIF1 MOL. WT. 36.5 kDa (6xHis tag fused to C-terminus with GS linker) SOURCE: HEK 293 cells PURITY: >95% SDS - PAGE FORM: Lyophilized FORMULATION: Lyophilized from 0.22 μm filtered PBS (pH 7.4) with 5% trehalose RECONSTITUTION: Centrifuge the vial prior to opening. Reconstitute in sterile PBS (pH 7.4). Do not vortex. STORAGE CONDITIONS: Store at -20°C. Once reconstituted, aliquot and store at -20°C or -70°C. Avoid repeated freezing and thawing cycles. DESCRIPTION: Trophoblast glycoprotein, also known as TPBG or 5T4, is a human cell surface protein encoded by a TPBG gene and an antagonist of the of Wnt/β-catenin signaling pathway. TPBG/5T4 is rarely expressed in normal adult tissues, but is present at high levels in placenta and in most common tumors, typically more than 80% of carcinomas of the kidney, breast, colon, prostate, and ovary. Due to its selective expression, it is often used as a prognostic aid in cancer cases. Recently, it has been shown that TPBG inhibits Wnt/β-catenin signaling, a signaling system central to many developmental and pathological processes and therefore serves as a therapeutic target of several anticancer agents currently in clinical development. AMINO ACID SEQUENCE: Ser 32 – Ser 355 SDS-PAGE (4-20%) of Recombinant TPBG: The recombinant protein is loaded under reducing (R) conditions and stained with Coomassie Blue. -
Trophoblast Glycoprotein Is a Marker for Efficient Sorting of Ventral
www.nature.com/npjparkd ARTICLE OPEN Trophoblast glycoprotein is a marker for efficient sorting of ventral mesencephalic dopaminergic precursors derived from human pluripotent stem cells Jeong-Eun Yoo1,7, Dongjin R. Lee1,7, Sanghyun Park1,2,7, Hye-Rim Shin1, Kun Gu Lee3, Dae-Sung Kim4, Mi-Young Jo5, Jang-Hyeon Eom5, ✉ ✉ Myung Soo Cho5, Dong-Youn Hwang3 and Dong-Wook Kim 1,2,6 Successful cell therapy for Parkinson’s disease (PD) requires large numbers of homogeneous ventral mesencephalic dopaminergic (vmDA) precursors. Enrichment of vmDA precursors via cell sorting is required to ensure high safety and efficacy of the cell therapy. Here, using LMX1A-eGFP knock-in reporter human embryonic stem cells, we discovered a novel surface antigen, trophoblast glycoprotein (TPBG), which was preferentially expressed in vmDA precursors. TPBG-targeted cell sorting enriched FOXA2+LMX1A+ vmDA precursors and helped attain efficient behavioral recovery of rodent PD models with increased numbers of TH+, NURR1+, and PITX3+ vmDA neurons in the grafts. Additionally, fewer proliferating cells were detected in TPBG+ cell-derived grafts than in TPBG− cell-derived grafts. Our approach is an efficient way to obtain enriched bona fide vmDA precursors, which could open a new avenue for effective PD treatment. npj Parkinson’s Disease (2021) 7:61 ; https://doi.org/10.1038/s41531-021-00204-8 1234567890():,; INTRODUCTION In this study, we discovered a novel surface marker, trophoblast + Parkinson’s disease (PD) is one of the most suitable neurodegen- glycoprotein (TPBG), which could be used to enrich LMX1A erative disorders for cell-based therapy due to the focal vmDA precursors for cell transplantation. -
Morphological Cell Profiling of SARS-Cov-2 Infection Identifies Drug Repurposing Candidates for COVID-19
Morphological cell profiling of SARS-CoV-2 infection identifies drug repurposing candidates for COVID-19 Carmen Mirabellia,1, Jesse W. Wotringb,c,1, Charles J. Zhangb,c,2, Sean M. McCartyb,2, Reid Fursmidtc,d,2, Carla D. Prettoc, Yuanyuan Qiaoe,f, Yuping Zhange,f, Tristan Frumg, Namrata S. Kadambic, Anya T. Aminc, Teresa R. O’Mearaa, Jason R. Spencec,g, Jessie Huangh,i,j, Konstantinos D. Alysandratosh,i,j, Darrell N. Kottonh,i,j, Samuel K. Handelmanc,d, Christiane E. Wobusa, Kevin J. Weatherwaxd,k,l, George A. Mashourd,k,m, Matthew J. O’Mearan,3, Arul M. Chinnaiyane,f,o,p,q,3,4, and Jonathan Z. Sextonb,c,d,k,3,4 aDepartment of Microbiology and Immunology, University of Michigan Medical School, Ann Arbor, MI 48109; bDepartment of Medicinal Chemistry, College of Pharmacy, University of Michigan, Ann Arbor, MI 48109; cDepartment of Internal Medicine, Division of Gastroenterology and Hepatology, Michigan Medicine at the University of Michigan, Ann Arbor, MI 48109; dCenter for Drug Repurposing, University of Michigan, Ann Arbor, MI 48109; eMichigan Center for Translational Pathology, University of Michigan, Ann Arbor, MI 48109; fDepartment of Pathology, University of Michigan, Ann Arbor, MI 48109; gDepartment of Cell and Developmental Biology, University of Michigan, Ann Arbor, MI 48109; hCenter for Regenerative Medicine, Boston University and Boston Medical Center, Boston, MA 02118; iPulmonary Center, Boston University School of Medicine, Boston, MA 02118; jDepartment of Medicine, Boston University School of Medicine, Boston, MA 02118; kMichigan -
A Genomic View of Estrogen Actions in Human Breast Cancer Cells by Expression Profiling of the Hormone-Responsive Transcriptome
719 A genomic view of estrogen actions in human breast cancer cells by expression profiling of the hormone-responsive transcriptome Luigi Cicatiello1, Claudio Scafoglio1, Lucia Altucci1, Massimo Cancemi1, Guido Natoli1, Angelo Facchiano2, Giovanni Iazzetti3, Raffaele Calogero4, Nicoletta Biglia6, Michele De Bortoli5,7, Christian Sfiligoi7, Piero Sismondi6,7, Francesco Bresciani1 and Alessandro Weisz1 1Dipartimento di Patologia generale, Seconda Università degli Studi di Napoli, Vico L. De Crecchio 7, 80138 Napoli, Italy 2Istituto di Scienze dell’Alimentazione del Consiglio Nazionale delle Ricerche, Avellino, Italy 3Dipartimento di Genetica, Biologia generale e molecolare, Università di Napoli ‘Federico II’, Napoli, Italy 4Dipartimento di Scienze cliniche e biologiche, Università degli Studi di Torino, Torino, Italy 5Dipartimento di Scienze oncologiche, Università degli Studi di Torino, Torino, Italy 6Dipartimento di Discipline ostetriche e ginecologiche, Università degli Studi di Torino, Torino, Italy 7Laboratorio di Ginecologia oncologica, Istituto per la Ricerca e la Cura del Cancro, Candiolo, Italy (Requests for offprints should be addressed to A Weisz; Email: [email protected]) Abstract Estrogen controls key cellular functions of responsive cells including the ability to survive, replicate, communicate and adapt to the extracellular milieu. Changes in the expression of 8400 genes were monitored here by cDNA microarray analysis during the first 32 h of human breast cancer (BC) ZR-75·1 cell stimulation with a mitogenic dose of 17-estradiol, a timing which corresponds to completion of a full mitotic cycle in hormone-stimulated cells. Hierarchical clustering of 344 genes whose expression either increases or decreases significantly in response to estrogen reveals that the gene expression program activated by the hormone in these cells shows 8 main patterns of gene activation/inhibition. -
Rab11b-Mediated Integrin Recycling Promotes Brain Metastatic
bioRxiv preprint doi: https://doi.org/10.1101/666750; this version posted June 11, 2019. The copyright holder for this preprint (which was not 6/10/2019 certified by peerRab11b-mediated review) is the integrinauthor/funder. recycling All promotes rights reserved. brain metastatic No reuse adaptation allowed and without outgrowth permission. - Google Docs Rab11bmediated integrin recycling promotes brain metastatic adaptation and outgrowth Erin N. Howe 1, 2 , Miranda D. Burnette 2,3,8 , Melanie E. Justice 2,4 , James W. Clancy 1 , Ian H. Guldner 1,2 , Patricia M. Schnepp 1, 2, 9 , Victoria Hendrick 5 , Uma K. Aryal 5 , Alicia T. Specht 2,6, , Jun Li 2,6 , Crislyn D’SouzaSchorey 1,2 , Jeremiah Z. Zartman 2,3 , and Siyuan Zhang 1, 2, 7, * Affiliations: 1. Department of Biological Sciences, University of Notre Dame, Notre Dame, IN, USA 2. Mike and Josie Harper Cancer Research Institute, University of Notre Dame, 1234 N. Notre Dame Avenue, South Bend, IN, USA 3. Department of Chemical and Biomolecular Engineering, University of Notre Dame, Notre Dame, IN, USA 4. Department of Chemistry and Biochemistry, University of Notre Dame, Notre Dame, IN, USA 5. Purdue Proteomics Facility, Bindley Bioscience Center, Discovery Park, Purdue University, West Lafayette, IN, USA 6. Department of Applied and Computational Mathematics and Statistics, University of Notre Dame, Notre Dame, IN, USA 7. Indiana University Melvin and Bren Simon Cancer Center, Indianapolis, IN, USA 8. Present address: Organogenesis, Birmingham, AL, USA 9. Present address: Department of Urology, University of Michigan Medical School, Ann Arbor, MI, USA * Correspondence: [email protected] Manuscript Summary: Rab11b upregulation in the brain microenvironment promotes recycling of cargo proteins required for breast cancer brain metastasis, including increased surface expression of integrin β1, which allows brain extracellular matrix attachment and mechanotransduction.