<I>Clostridium Thermocellum</I> for Improved Cellulosic Etha
Total Page:16
File Type:pdf, Size:1020Kb
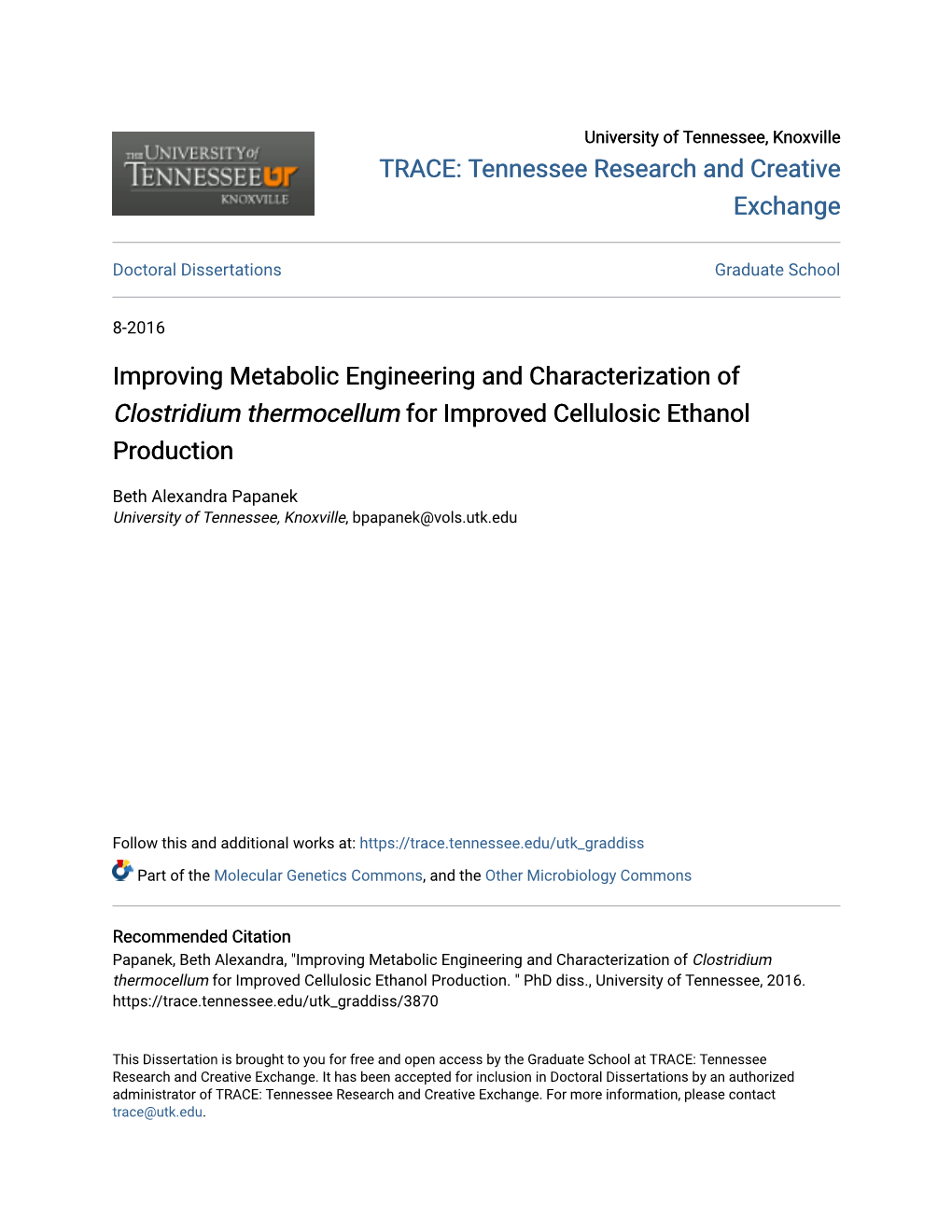
Load more
Recommended publications
-
CO2-Fixing One-Carbon Metabolism in a Cellulose-Degrading Bacterium
CO2-fixing one-carbon metabolism in a cellulose- degrading bacterium Clostridium thermocellum Wei Xionga, Paul P. Linb, Lauren Magnussona, Lisa Warnerc, James C. Liaob,d, Pin-Ching Manessa,1, and Katherine J. Choua,1 aBiosciences Center, National Renewable Energy Laboratory, Golden, CO 80401; bDepartment of Chemical and Biomolecular Engineering, University of California, Los Angeles, CA 90095; cNational Bioenergy Center, National Renewable Energy Laboratory, Golden, CO 80401; and dAcademia Sinica, Taipei City, Taiwan 115 Edited by Lonnie O. Ingram, University of Florida, Gainesville, FL, and approved September 23, 2016 (received for review April 6, 2016) Clostridium thermocellum can ferment cellulosic biomass to for- proposed pathway involving CO2 utilization in C. thermocellum is mate and other end products, including CO2. This organism lacks the malate shunt and its variant pathway (7, 14), in which CO2 is formate dehydrogenase (Fdh), which catalyzes the reduction of first incorporated by phosphoenolpyruvate carboxykinase (PEPCK) 13 CO2 to formate. However, feeding the bacterium C-bicarbonate to form oxaloacetate and then is released either by malic enzyme or and cellobiose followed by NMR analysis showed the production via oxaloacetate decarboxylase complex, yielding pyruvate. How- of 13C-formate in C. thermocellum culture, indicating the presence ever, other pathways capable of carbon fixation may also exist but of an uncharacterized pathway capable of converting CO2 to for- remain uncharacterized. mate. Combining genomic and experimental data, we demon- In recent years, isotopic tracer experiments followed by mass strated that the conversion of CO2 to formate serves as a CO2 spectrometry (MS) (15, 16) or NMR measurements (17) have entry point into the reductive one-carbon (C1) metabolism, and emerged as powerful tools for metabolic analysis. -
Specialized Cell Surface Structures in Cellulolytic Bacteria RAPHAEL LAMED,'* JENNY NAIMARK,' ELY MORGENSTERN,' and EDWARD A
JOURNAL OF BACTERIOLOGY, Aug. 1987, p. 3792-3800 Vol. 169, No. 8 0021-9193/87/083792-09$02.00/0 Copyright © 1987, American Society for Microbiology Specialized Cell Surface Structures in Cellulolytic Bacteria RAPHAEL LAMED,'* JENNY NAIMARK,' ELY MORGENSTERN,' AND EDWARD A. BAYER2 Center for Biotechnology, George S. Wise Faculty ofLife Sciences, Tel Aviv University, Ramat Aviv,1 and Department of Biophysics, The Weizmann Institute of Science, Rehovot,2 Israel Received 30 March 1987/Accepted 22 May 1987 The cell surface topology of various gram-negative and -positive, anaerobic and aerobic, mesophilic and thermophilic, cellulolytic and noncellulolytic bacteria was investigated by scanning electron microscopic visualization using cationized ferritin. Characterisitic protuberant structures were observed on cells of all cellulolytic strains. These structures appeared to be directly related to the previously described exocellular cellulase-containing polycellulosomes of Clostridium thermocellum YS (E. A. Bayer and R. Lamed, J. Bacteriol. 167:828-836, 1986). Immunochemical evidence and lectin-binding studies suggested a further correlation on the molecular level among cellulolytic bacteria. The results indicate that such cell surface cellulase-containing structures may be of general consequence to the bacterial interaction with and degradation of cellulose. We have recently demonstrated by various means that MATERIALS AND METHODS cellulolytic enzymes of the gram-positive, thermophilic anaerobe Clostridium thermocellum are organized into a Organisms and culture conditions. The bacterial strains distinct multisubunit complex which we have called the used in this study are listed in Table 1. All strains of C. "cellulosome" (2, 16, 17). The cellulosome in this organism thermocellum were cultivated under anaerobic conditions at appears both in an extracellular and in a cell-associated 60°C in cellobiose-containing medium as described in refer- form. -
Supporting Information
Supporting Information Lozupone et al. 10.1073/pnas.0807339105 SI Methods nococcus, and Eubacterium grouped with members of other Determining the Environmental Distribution of Sequenced Genomes. named genera with high bootstrap support (Fig. 1A). One To obtain information on the lifestyle of the isolate and its reported member of the Bacteroidetes (Bacteroides capillosus) source, we looked at descriptive information from NCBI grouped firmly within the Firmicutes. This taxonomic error was (www.ncbi.nlm.nih.gov/genomes/lproks.cgi) and other related not surprising because gut isolates have often been classified as publications. We also determined which 16S rRNA-based envi- Bacteroides based on an obligate anaerobe, Gram-negative, ronmental surveys of microbial assemblages deposited near- nonsporulating phenotype alone (6, 7). A more recent 16S identical sequences in GenBank. We first downloaded the gbenv rRNA-based analysis of the genus Clostridium defined phylo- files from the NCBI ftp site on December 31, 2007, and used genetically related clusters (4, 5), and these designations were them to create a BLAST database. These files contain GenBank supported in our phylogenetic analysis of the Clostridium species in the HGMI pipeline. We thus designated these Clostridium records for the ENV database, a component of the nonredun- species, along with the species from other named genera that dant nucleotide database (nt) where 16S rRNA environmental cluster with them in bootstrap supported nodes, as being within survey data are deposited. GenBank records for hits with Ͼ98% these clusters. sequence identity over 400 bp to the 16S rRNA sequence of each of the 67 genomes were parsed to get a list of study titles Annotation of GTs and GHs. -
New Insights Into Clostridia Through Comparative Analyses of Their 40 Genomes
Bioenerg. Res. DOI 10.1007/s12155-014-9486-9 New Insights into Clostridia Through Comparative Analyses of Their 40 Genomes Chuan Zhou & Qin Ma & Xizeng Mao & Bingqiang Liu & Yanbin Yin & Ying Xu # Springer Science+Business Media New York 2014 Abstract The Clostridium genus of bacteria contains the gene groups are shared by all the strains, and the strain- most widely studied biofuel-producing organisms such as specific gene pool consists of 22,668 genes, which is consis- Clostridium thermocellum and also some human pathogens, tent with the fact that these bacteria live in very diverse plus a few less characterized strains. Here, we present a environments and have evolved a very large number of comparative genomic analysis of 40 fully sequenced clostrid- strain-specific genes to adapt to different environments. ial genomes, paying a particular attention to the biomass Across the 40 genomes, 1.4–5.8 % of genes fall into the degradation ones. Our analysis indicates that some of the carbohydrate active enzyme (CAZyme) families, and 20 out Clostridium botulinum strains may have been incorrectly clas- of the 40 genomes may encode cellulosomes with each ge- sified in the current taxonomy and hence should be renamed nome having 1 to 76 genes bearing the cellulosome-related according to the 16S ribosomal RNA (rRNA) phylogeny. A modules such as dockerins and cohesins. A phylogenetic core-genome analysis suggests that only 169 orthologous footprinting analysis identified cis-regulatory motifs that are enriched in the promoters of the CAZyme genes, giving rise to 32 statistically significant motif candidates. Keywords Clostridium . Comparative genomics . -
Clostridium Amazonitimonense, Clostridium Me
ORIGINAL ARTICLE Taxonogenomic description of four new Clostridium species isolated from human gut: ‘Clostridium amazonitimonense’, ‘Clostridium merdae’, ‘Clostridium massilidielmoense’ and ‘Clostridium nigeriense’ M. T. Alou1, S. Ndongo1, L. Frégère1, N. Labas1, C. Andrieu1, M. Richez1, C. Couderc1, J.-P. Baudoin1, J. Abrahão2, S. Brah3, A. Diallo1,4, C. Sokhna1,4, N. Cassir1, B. La Scola1, F. Cadoret1 and D. Raoult1,5 1) Aix-Marseille Université, Unité de Recherche sur les Maladies Infectieuses et Tropicales Emergentes, UM63, CNRS 7278, IRD 198, INSERM 1095, Marseille, France, 2) Laboratório de Vírus, Departamento de Microbiologia, Universidade Federal de Minas Gerais, Belo Horizonte, Minas Gerais, Brazil, 3) Hopital National de Niamey, BP 247, Niamey, Niger, 4) Campus Commun UCAD-IRD of Hann, Route des pères Maristes, Hann Maristes, BP 1386, CP 18524, Dakar, Senegal and 5) Special Infectious Agents Unit, King Fahd Medical Research Center, King Abdulaziz University, Jeddah, Saudi Arabia Abstract Culturomics investigates microbial diversity of the human microbiome by combining diversified culture conditions, matrix-assisted laser desorption/ionization time-of-flight mass spectrometry and 16S rRNA gene identification. The present study allowed identification of four putative new Clostridium sensu stricto species: ‘Clostridium amazonitimonense’ strain LF2T, ‘Clostridium massilidielmoense’ strain MT26T, ‘Clostridium nigeriense’ strain Marseille-P2414T and ‘Clostridium merdae’ strain Marseille-P2953T, which we describe using the concept of taxonogenomics. We describe the main characteristics of each bacterium and present their complete genome sequence and annotation. © 2017 Published by Elsevier Ltd. Keywords: ‘Clostridium amazonitimonense’, ‘Clostridium massilidielmoense’, ‘Clostridium merdae’, ‘Clostridium nigeriense’, culturomics, emerging bacteria, human microbiota, taxonogenomics Original Submission: 18 August 2017; Revised Submission: 9 November 2017; Accepted: 16 November 2017 Article published online: 22 November 2017 intestine [1,4–6]. -
Development of a Genome-Scale Metabolic Model of Clostridium Thermocellum and Its Applications for Integration of Multi-Omics Datasets and Computational Strain Design
ORIGINAL RESEARCH published: 21 August 2020 doi: 10.3389/fbioe.2020.00772 Development of a Genome-Scale Metabolic Model of Clostridium thermocellum and Its Applications for Integration of Multi-Omics Datasets and Computational Strain Design Sergio Garcia 1,2, R. Adam Thompson 2,3,4†, Richard J. Giannone 2,5, Satyakam Dash 2,6, 2,6 1,2,3,4 Edited by: Costas D. Maranas and Cong T. Trinh * Young-Mo Kim, 1 2 Pacific Northwest National Laboratory Department of Chemical and Biomolecular Engineering, The University of Tennessee, Knoxville, TN, United States, Center 3 (DOE), United States for Bioenergy Innovation, Oak Ridge National Laboratory, Oak Ridge, TN, United States, Bredesen Center for Interdisciplinary Research and Graduate Education, The University of Tennessee, Knoxville, TN, United States, 4 Oak Ridge Reviewed by: National Laboratory, Oak Ridge, TN, United States, 5 Chemical Sciences Division, Oak Ridge National Laboratory, Oak Ridge, Karsten Zengler, TN, United States, 6 Department of Chemical Engineering, The Pennsylvania State University, University Park, PA, University of California, San Diego, United States United States Esteban Marcellin, The University of Queensland, Solving environmental and social challenges such as climate change requires a shift Australia from our current non-renewable manufacturing model to a sustainable bioeconomy. *Correspondence: To lower carbon emissions in the production of fuels and chemicals, plant biomass Cong T. Trinh [email protected] feedstocks can replace petroleum using microorganisms as biocatalysts. The anaerobic thermophile Clostridium thermocellum is a promising bacterium for bioconversion due †Present address: R. Adam Thompson, to its capability to efficiently degrade lignocellulosic biomass. However, the complex Quantitative Translational metabolism of C. -
Human Microbiota Reveals Novel Taxa and Extensive Sporulation Hilary P
OPEN LETTER doi:10.1038/nature17645 Culturing of ‘unculturable’ human microbiota reveals novel taxa and extensive sporulation Hilary P. Browne1*, Samuel C. Forster1,2,3*, Blessing O. Anonye1, Nitin Kumar1, B. Anne Neville1, Mark D. Stares1, David Goulding4 & Trevor D. Lawley1 Our intestinal microbiota harbours a diverse bacterial community original faecal sample and the cultured bacterial community shared required for our health, sustenance and wellbeing1,2. Intestinal an average of 93% of raw reads across the six donors. This overlap was colonization begins at birth and climaxes with the acquisition of 72% after de novo assembly (Extended Data Fig. 2). Comparison to a two dominant groups of strict anaerobic bacteria belonging to the comprehensive gene catalogue that was derived by culture-independent Firmicutes and Bacteroidetes phyla2. Culture-independent, genomic means from the intestinal microbiota of 318 individuals4 found that approaches have transformed our understanding of the role of the 39.4% of the genes in the larger database were represented in our cohort human microbiome in health and many diseases1. However, owing and 73.5% of the 741 computationally derived metagenomic species to the prevailing perception that our indigenous bacteria are largely identified through this analysis were also detectable in the cultured recalcitrant to culture, many of their functions and phenotypes samples. remain unknown3. Here we describe a novel workflow based on Together, these results demonstrate that a considerable proportion of targeted phenotypic culturing linked to large-scale whole-genome the bacteria within the faecal microbiota can be cultured with a single sequencing, phylogenetic analysis and computational modelling that growth medium. -
Ruminococcal Cellulosomes: Molecular Lego to Deconstruct Microcrystalline Cellulose in Human Gut Gurvan Michel
Ruminococcal cellulosomes: molecular Lego to deconstruct microcrystalline cellulose in human gut Gurvan Michel To cite this version: Gurvan Michel. Ruminococcal cellulosomes: molecular Lego to deconstruct microcrystalline cellulose in human gut. Environmental Microbiology, Society for Applied Microbiology and Wiley-Blackwell, 2015, 17 (9), pp.3113-3115. 10.1111/1462-2920.12920. hal-02137960 HAL Id: hal-02137960 https://hal.archives-ouvertes.fr/hal-02137960 Submitted on 23 May 2019 HAL is a multi-disciplinary open access L’archive ouverte pluridisciplinaire HAL, est archive for the deposit and dissemination of sci- destinée au dépôt et à la diffusion de documents entific research documents, whether they are pub- scientifiques de niveau recherche, publiés ou non, lished or not. The documents may come from émanant des établissements d’enseignement et de teaching and research institutions in France or recherche français ou étrangers, des laboratoires abroad, or from public or private research centers. publics ou privés. 1 Highlights on: Ben David et al (2015) Ruminococcal cellulosome systems from rumen to 2 human. Environmental Microbiology 3 4 5 Ruminococcal cellulosomes: molecular Lego to deconstruct microcrystalline cellulose in 6 human gut 7 8 Gurvan Michel 9 10 Sorbonne Université, UPMC Univ Paris 06, CNRS, UMR 8227, Integrative Biology of 11 Marine Models, Station Biologique de Roscoff, CS 90074, F-29688, Roscoff cedex, Bretagne, 12 France 13 For correspondence: Station Biologique de Roscoff, Place Georges Teissier, 29688, Roscoff, 14 Bretagne, France. Tel.: 33-298-29-23-30; Fax: 33-298-29-23-24; E-mail: gurvan.michel@sb- 15 roscoff.fr. 16 1 Plants (and also algae in East Asian cuisine) constitute the bases of human diet. -
Clostridium Cellulolyticum: Model Organism of Mesophilic Cellulolytic Clostridia
FEMS Microbiology Reviews 29 (2005) 741–764 www.fems-microbiology.org Clostridium cellulolyticum: model organism of mesophilic cellulolytic clostridia Mickae¨l Desvaux * Institute for Biomedical Research, The University of Birmingham – The Medical School, Vincent Drive, Edgbaston, Birmingham B15 2TT, UK Received 26 November 2003; received in revised form 27 April 2004; accepted 1 November 2004 First published online 7 December 2004 Abstract Clostridium cellulolyticum ATCC 35319 is a non-ruminal mesophilic cellulolytic bacterium originally isolated from decayed grass. As with most truly cellulolytic clostridia, C. cellulolyticum possesses an extracellular multi-enzymatic complex, the cellulosome. The catalytic components of the cellulosome release soluble cello-oligosaccharides from cellulose providing the primary carbon sub- strates to support bacterial growth. As most cellulolytic bacteria, C. cellulolyticum was initially characterised by limited carbon con- sumption and subsequent limited growth in comparison to other saccharolytic clostridia. The first metabolic studies performed in batch cultures suggested nutrient(s) limitation and/or by-product(s) inhibition as the reasons for this limited growth. In most recent investigations using chemostat cultures, metabolic flux analysis suggests a self-intoxication of bacterial metabolism resulting from an inefficiently regulated carbon flow. The investigation of C. cellulolyticum physiology with cellobiose, as a model of soluble cellod- extrin, and with pure cellulose, as a carbon source more -
Cellulose Fermentation by Clostridium Thermocellum and a Mixed Consortium in an Automated Repetitive Batch Reactor ⇑ Parker T
Bioresource Technology 155 (2014) 50–56 Contents lists available at ScienceDirect Bioresource Technology journal homepage: www.elsevier.com/locate/biortech Cellulose fermentation by Clostridium thermocellum and a mixed consortium in an automated repetitive batch reactor ⇑ Parker T. Reed, Javier A. Izquierdo 1, Lee R. Lynd Thayer School of Engineering, Dartmouth College, Hanover, NH 03755, United States BioEnergy Science Center, Oak Ridge National Laboratory, Oak Ridge, TN 37831, United States highlights An automated repetitive batch fermentation system was developed. A strong correlation was observed between CO2 produced and cellulose consumed. C. thermocellum and a mixed consortium exhibit accelerated substrate utilization. Similar numbers of cycles were needed to reach maximum CO2 production rates. Maximum CO2 production rates and cycle times were very similar for both cultures. article info abstract Article history: An automated repetitive batch fermentation system was developed to facilitate the study of microbial Received 4 October 2013 cellulose utilization. The system was operated with Avicel as the carbon source and either Clostridium Received in revised form 10 December 2013 thermocellum ATCC 27405 or a consortium enriched from compost as inocula. Multiple cycles of growth Accepted 12 December 2013 medium addition, incubation, and medium removal were performed with each inoculum. Removal and Available online 25 December 2013 addition of media were automatically initiated when CO2 production fell to 90% of the cycle’s peak. A strong correlation was observed between CO2 production and cellulose consumption, suggesting that Keywords: the online signal was a good proxy for substrate utilization. Both cultures exhibited accelerated substrate Automated batch fermentation utilization and a decrease in cycle time. -
Clostridium Thermocellum from Icelandic Hot Springs ANDREW C
INTERNATIONALJOURNAL OF SYSTEMATICBACTERIOLOGY, Jan. 1988, p. 119-121 Vol. 38, No. 1 0020-7713/88/010119-03$02.00/0 Copyright 0 1988, International Union of Microbiological Societies Isolation and Properties of Clostridium thermocellum from Icelandic Hot Springs ANDREW C. STAINTHORPE AND RALPH A. D. WILLIAMS* Biochemistry Department, The London Hospital Medical College, London El 2AD, United Kingdom Six strains of Clostridium thermocellum were isolated from hot springs near Hveragerthi, Iceland, and compared with strains of this and other species of clostridia. Deoxyribonucleic acid homology showed that the strains of this species formed a genetic species distinct from all others. The anaerobic thermophile Clostridium thermocellum has All strains were gram variable throughout growth and were often been used for the simultaneous saccharification and lysed by 3% potassium hydroxide (6). Zones of cellulose fermentation of lignocellulosic biomasses (18, 27). Cellulo- clearing were distinct in 1 to 2 days at 60°C. Terminal spores lytic bacteria have been isolated from hot springs (21, 24) (1.2 to 2.0 by 2.0 to 2.2 pm) were round to oval. All strains and decomposing biomasses (22). Strains with broad sub- were obligate anaerobes and required yeast extract. All strate ranges that are of potential biotechnological use have strains fermented cellulose, D-cellobiose, D-glucose, and been described (7, 13, 15, 23). C. thermocellum cultures are sorbitol. Strains B2, A2, H20, and G2 fermented D-fructose, often associated with contaminants (26), and identification of and strains B2 and H20 also fermented raffinose and lactose the species is hampered by differences in the descriptions, weakly. -
Fermentation of Cellulosic Substrates in Batch and Continuous Culture by Clostridium Thermocellum
Dartmouth College Dartmouth Digital Commons Open Dartmouth: Peer-reviewed articles by Dartmouth faculty Faculty Work 9-20-1989 Fermentation of Cellulosic Substrates in Batch and Continuous Culture by Clostridium thermocellum Lee R. Lynd Dartmouth College Hans E. Grethlein Dartmouth College Richard H. Wolkin Dartmouth College Follow this and additional works at: https://digitalcommons.dartmouth.edu/facoa Part of the Biochemistry, Biophysics, and Structural Biology Commons Dartmouth Digital Commons Citation Lynd, Lee R.; Grethlein, Hans E.; and Wolkin, Richard H., "Fermentation of Cellulosic Substrates in Batch and Continuous Culture by Clostridium thermocellum" (1989). Open Dartmouth: Peer-reviewed articles by Dartmouth faculty. 502. https://digitalcommons.dartmouth.edu/facoa/502 This Article is brought to you for free and open access by the Faculty Work at Dartmouth Digital Commons. It has been accepted for inclusion in Open Dartmouth: Peer-reviewed articles by Dartmouth faculty by an authorized administrator of Dartmouth Digital Commons. For more information, please contact [email protected]. APPLIED AND ENVIRONMENTAL MICROBIOLOGY, Dec. 1989, p. 3131-3139 Vol. 55, No. 12 0099-2240/89/123131-09$02.00/0 Copyright C) 1989, American Society for Microbiology Fermentation of Cellulosic Substrates in Batch and Continuous Culture by Clostridium thermocellum LEE RYBECK LYND,* HANS E. GRETHLEIN,t AND RICHARD H. WOLKINt Thayer School of Engineering, Dartmouth College, Hanover, New Hampshire 03755 Received 19 December 1988/Accepted 20 September 1989 Fermentation of dilute-acid-pretreated mixed hardwood and Avicel by Clostridium thermocellum was compared in batch and continuous cultures. Maximum specific growth rates per hour obtained on cellulosic substrates were 0.1 in batch culture and >0.13 in continuous culture.