Physical Realization of Non-Radiative Wireless Power Transmission Using Zenneck Waves
Total Page:16
File Type:pdf, Size:1020Kb
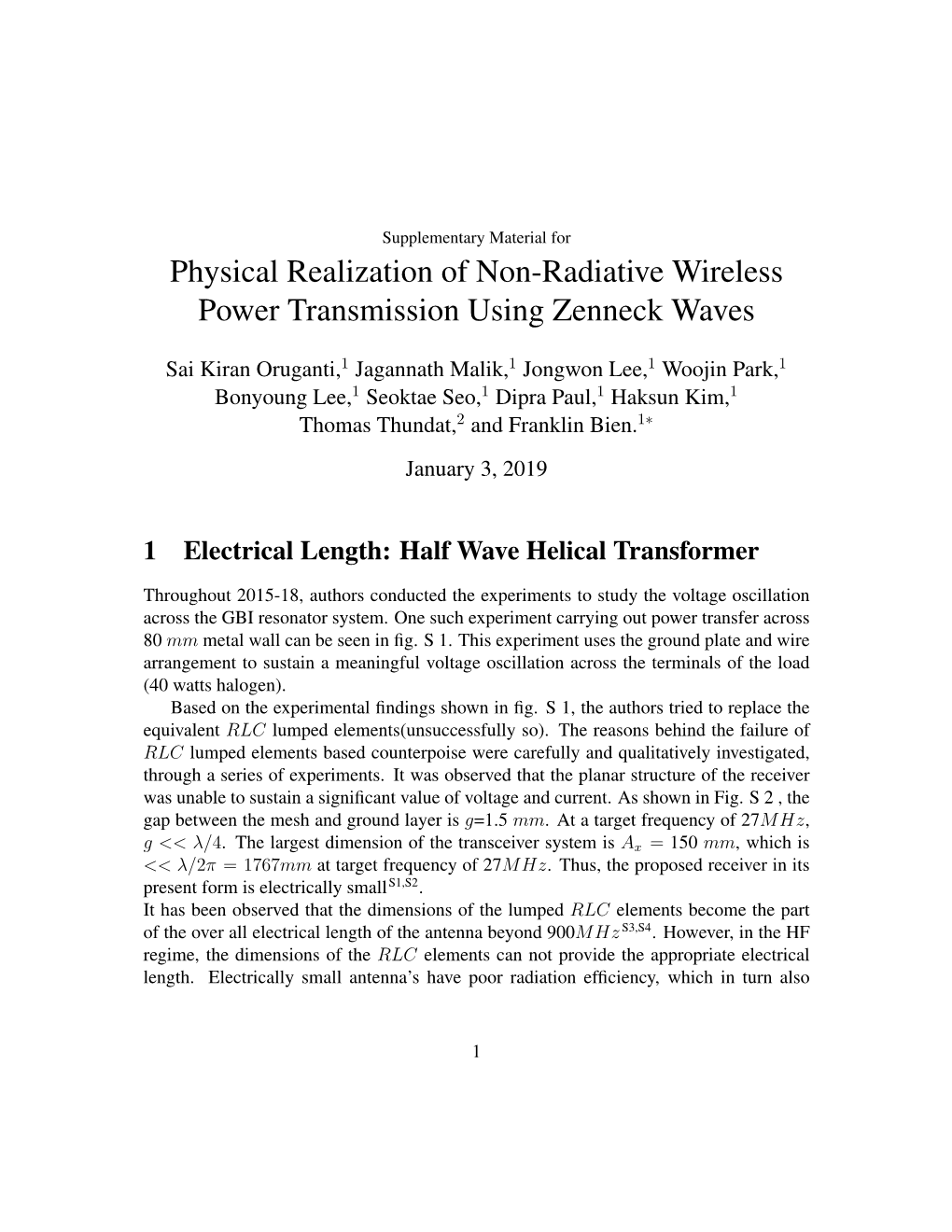
Load more
Recommended publications
-
Compact Bilateral Single Conductor Surface Wave Transmission Line
Compact bilateral single conductor Regarded as half mode CPW, a series of slotlines have been proposed whose characteristic impedance is easy to control [7]. The bilateral surface wave transmission line slotline with 50 ohm characteristic impedance is designed as shown in Fig. 2. The copper layers on both sides of the substrate are connected by Zhixia Xu, Shunli Li, Hongxin Zhao, Leilei Liu and the metalized via arrays, and the two via arrays can increase the perunit- Xiaoxing Yin length capacitance and decrease characteristic impedance to 50 ohm which is usually quite high for a conventional slotline. The height of via A compact bilateral single conductor surface wave transmission line h is 1.524 mm, the slot S is 0.3 mm; the space between via arrays d is (TL) is proposed, converting the quasi-transverse electromagnetic 0.4 mm; the space between neighbour vias a is 1 mm. (QTEM) mode of low characteristic impedance slotline into the As a single conductor TL, bilateral corrugated metallic strips are transverse magnetic (TM) mode of single-conductor TL. The propagation constant of the proposed TL is decided by geometric printed on both sides of the substrate and connected by via arrays the parameters of the periodic corrugated structure. Compared to configuration is shown in Fig. 3a. To excite the transverse magnetic conventional transitions between coplanar waveguide (CPW) and (TM) mode, the surface mode of the corrugated strip, from the quasi- single-conductor TLs, such as Goubau line (G-Line) and surface transverse electromagnetic (QTEM) mode, the mode of slotline, we plasmons TL, the proposed structure halves the size and this feature propose a compact bilateral transition structure, as shown in Fig. -
W5GI MYSTERY ANTENNA (Pdf)
W5GI Mystery Antenna A multi-band wire antenna that performs exceptionally well even though it confounds antenna modeling software Article by W5GI ( SK ) The design of the Mystery antenna was inspired by an article written by James E. Taylor, W2OZH, in which he described a low profile collinear coaxial array. This antenna covers 80 to 6 meters with low feed point impedance and will work with most radios, with or without an antenna tuner. It is approximately 100 feet long, can handle the legal limit, and is easy and inexpensive to build. It’s similar to a G5RV but a much better performer especially on 20 meters. The W5GI Mystery antenna, erected at various heights and configurations, is currently being used by thousands of amateurs throughout the world. Feedback from users indicates that the antenna has met or exceeded all performance criteria. The “mystery”! part of the antenna comes from the fact that it is difficult, if not impossible, to model and explain why the antenna works as well as it does. The antenna is especially well suited to hams who are unable to erect towers and rotating arrays. All that’s needed is two vertical supports (trees work well) about 130 feet apart to permit installation of wire antennas at about 25 feet above ground. The W5GI Multi-band Mystery Antenna is a fundamentally a collinear antenna comprising three half waves in-phase on 20 meters with a half-wave 20 meter line transformer. It may sound and look like a G5RV but it is a substantially different antenna on 20 meters. -
California State University, Northridge
CALIFORNIA STATE UNIVERSITY, NORTHRIDGE Design of a 5.8 GHz Two-Stage Low Noise Amplifier A graduate project submitted in partial fulfillment of the requirements For the degree of Master of Science in Electrical Engineering By Yashika Parwath August 2020 The graduate project of Yashika Parwath is approved: Dr. John Valdovinos Date Dr. Jack Ou Date Dr. Brad Jackson, Chair Date California State University, Northridge ii Acknowledgement I would like to express my sincere gratitude to Dr. Brad Jackson for his unwavering support and mentorship that aided me to finish my master’s project. With his deep understanding of the subject and valuable inputs this design project has been quite a learning wheel expanding my knowledge horizons. I would also like to thank Dr. John Valdovinos and Dr. Jack Ou for being the esteemed members of the committee. iii Table of Contents Signature page ii Acknowledgement iii List of Figures v List of Tables vii Abstract viii Chapter 1: Introduction 1 1.1 Communication System 1 1.2 Low Noise Amplifier 2 1.3 Design Goals 2 Chapter 2: LNA Theory and Background 4 2.1 Introduction 4 2.2 Terminology 4 2.3 Design Procedure 10 Chapter 3: LNA Design Procedure 12 3.1 Transistor 12 3.2 S-Parameters 12 3.3 Stability 13 3.4 Noise and Noise Figure 16 3.5 Cascaded Noise Figure 16 3.6 Noise Circles 17 3.7 Unilateral Figure of Merit 18 3.8 Gain 20 Chapter 4: Source and Load Reflection Coefficient 23 4.1 Reflection Coefficient 23 4.2 Source Reflection Coefficient 24 4.3 Load Reflection Coefficient 26 Chapter 5: Impedance Matching -
Circuit and Method for Balun Compensation
Europaisches Patentamt J European Patent Office © Publication number: 0 644 605 A1 Office europeen des brevets EUROPEAN PATENT APPLICATION © Application number: 94112882.9 int. CI 6: H01P 5/10 @ Date of filing: 18.08.94 © Priority: 22.09.93 US 124875 © Applicant: MOTOROLA, INC. 1303 East Algonquin Road @ Date of publication of application: Schaumburg, IL 60196 (US) 22.03.95 Bulletin 95/12 @ Inventor: Kaltenecker, Robert S. © Designated Contracting States: 2719 S. Estrella Circle DE FR GB Mesa, Arizona 85202 (US) Inventor: Pfizenmayer, Henry L. 3318 E. Turquiose Avenue Phoenix, Arizona 85028 (US) Inventor: Wernett, Frederick C. 492 Kweo Trail Flagstaff, Arizona 86001 (US) © Representative: Hudson, Peter David et al Motorola European Intellectual Property Midpoint Alencon Link Basingstoke, Hampshire RG21 1PL (GB) © Circuit and method for balun compensation. 10 © A novel circuit and method for providing am- 14 plitude and phase compensation for a balun in order P1 P2 7o,Eo | Ox to provide first and second voltage signals that are 12 1 16 balanced has been provided. The compensation is I rrm < ^ achieved by an amplitude and phase com- adding P3 pensation circuit such as a transmission line (14) or 1 mm 18 o inductive (20) and capacitive (22) lumped elements CO in series with one of the ports of the balun on the balanced side. The and amplitude phase compensa- FIG. 1 tion circuit includes a characteristic impedance CO pa- rameter (Zo) and an electrical length parameter (Eo) that are optimized such that the amplitude difference between first and second voltage signals is mini- mized, while the magnitude of the phase difference between first and second voltage signals is maxi- mized. -
Design and Application of a New Planar Balun
DESIGN AND APPLICATION OF A NEW PLANAR BALUN Younes Mohamed Thesis Prepared for the Degree of MASTER OF SCIENCE UNIVERSITY OF NORTH TEXAS May 2014 APPROVED: Shengli Fu, Major Professor and Interim Chair of the Department of Electrical Engineering Hualiang Zhang, Co-Major Professor Hyoung Soo Kim, Committee Member Costas Tsatsoulis, Dean of the College of Engineering Mark Wardell, Dean of the Toulouse Graduate School Mohamed, Younes. Design and Application of a New Planar Balun. Master of Science (Electrical Engineering), May 2014, 41 pp., 2 tables, 29 figures, references, 21 titles. The baluns are the key components in balanced circuits such balanced mixers, frequency multipliers, push–pull amplifiers, and antennas. Most of these applications have become more integrated which demands the baluns to be in compact size and low cost. In this thesis, a new approach about the design of planar balun is presented where the 4-port symmetrical network with one port terminated by open circuit is first analyzed by using even- and odd-mode excitations. With full design equations, the proposed balun presents perfect balanced output and good input matching and the measurement results make a good agreement with the simulations. Second, Yagi-Uda antenna is also introduced as an entry to fully understand the quasi-Yagi antenna. Both of the antennas have the same design requirements and present the radiation properties. The arrangement of the antenna’s elements and the end-fire radiation property of the antenna have been presented. Finally, the quasi-Yagi antenna is used as an application of the balun where the proposed balun is employed to feed a quasi-Yagi antenna. -
Long Earthquake'waves
~ LONG EARTHQUAKE'WAVES Seisllloloo-istsLl are tuning their instnlments to record earth 1l10tiollS with periods of one Jninute to an hour and mllplitudes of less than .01 inch. These,,~aycs tell llluch about the earth's crust and HHltltle by Jack Oliver "lhe hi-H enthusiast who hitS strug· per cycle. A reI.ltlvely shorr earthquake earth'luake waves tmvel at .9 to 1.S 1 gled to ImprO\'e tlIe re;pollse of wave has a duration of 10 seconds. At miles per second, they lange in length hI:; e'jlllpment In the bass range at present the most infornl.1tive long waves from 10 mile" up to the 8,OOO-mde length .11 oUlld 10 c~'d(-s per ;econd wIll ha VI" a h,\v€ periods of 15 to 75 seconds from of the earth's ,hamete... TheIr amplitude, fellow-feeling for the selsmologi;t \vho CfEst to crest. \Vith more advanced in however, is of an entirely di/lerent 01 de.. : IS ,attempting to tune hiS installments to ,,11 uments seismologists hope sooo to one of these waves displaces a point on the longest earth'luake \\".\\'es. But where study 450-second waves. The longest so the sudace of the eal th at some dislil1lce IIl-n deals in cITIes per second, seisll1OIo" f.tr detected had ., penod of :3,400 sec from the shock by no more than a hun· gy measures its fre<luencle; 111 seconds onds-·nearly an hour! Since these long dredth of an inch, even when eXCited by t/) ~ ~ SUBTERRANEAN TOPOGRAPHY of the North American con· at which the depth of the boundary was established. -
A Confusing Sixer of Beer: Tales of Six Frothy Trademark Disputes
University of the Pacific Law Review Volume 52 Issue 4 Article 8 1-10-2021 A Confusing Sixer of Beer: Tales of Six Frothy Trademark Disputes Rebecca E. Crandall Attorney Follow this and additional works at: https://scholarlycommons.pacific.edu/uoplawreview Recommended Citation Rebecca E. Crandall, A Confusing Sixer of Beer: Tales of Six Frothy Trademark Disputes, 52 U. PAC. L. REV. 783 (2021). Available at: https://scholarlycommons.pacific.edu/uoplawreview/vol52/iss4/8 This Article is brought to you for free and open access by the Journals and Law Reviews at Scholarly Commons. It has been accepted for inclusion in University of the Pacific Law Review by an authorized editor of Scholarly Commons. For more information, please contact [email protected]. A Confusing Sixer of Beer: Tales of Six Frothy Trademark Disputes Rebecca E. Crandall* I. 2017 AT THE TTAB: COMMERCIAL IMPRESSION IN INSPIRE V. INNOVATION .. 784 II. 2013 IN KENTUCKY: CONFUSION WITH UPSIDE DOWN NUMBERS AND A DINGBAT STAR ...................................................................................... 787 III. 1960S IN GEORGIA: BEER AND CIGARETTES INTO THE SAME MOUTH ........ 790 IV. 2020 IN BROOKLYN: RELATED GOODS AS BETWEEN BEER AND BREWING KITS ....................................................................................... 792 V. 2015 IN TEXAS: TARNISHMENT IN REMEMBERING THE ALAMO .................. 795 VI. 2016 AT THE TTAB: LAWYERS AS THE PREDATORS ................................... 797 VII. CONCLUSION ............................................................................................ -
Surface Waves: What Are They? Why Are They Interesting?
SURFACE WAVES: WHAT ARE THEY? WHY ARE THEY INTERESTING? Janice Hendry Roke Manor Research Limited, Old Salisbury Lane, Romsey, SO51 0ZN, UK [email protected] ABSTRACT Surface waves have been known about for many decades and quite in-depth research took place up until ~1960s . Since then, little work has been done in this area. It is unclear why, however today we have more powerful modelling methods which may enable us to understand their use better. It is known that high frequency (HF) surface waves follow the terrain and this has been utilised in some cases such as Raytheon's HF surface wave radar (HFSWR) for detecting ships over-the-horizon. It is thought with some more insight and knowledge of this phenomenon, surface waves could be extremely useful for both military and civil use, from communications to agriculture. WHAT ARE SURFACE WAVES? Over the many decades that surface waves have been researched, some confusion has built up over exactly what constitutes a surface wave. This was discussed by James Wait in an IEE Transaction in 1965 1 and was even a matter of discussion at the General Assembly of International Union of Radio Science (URSI) held in London in 1960. It was concluded that “there is no neat definition which would encompass all forms of wave which could glide or be guided along an interface”. However, the definitions which have been settled upon for this paper are as follows: Surface Wave Region : The region of interest in which the surface wave propagates. Surface Wave : A surface wave is one that propagates along an interface between two different media without radiation; such radiation being construed to mean energy converted from the surface wave field to some other form. -
Sky-Wave Propagation
ANTENNA AND WAVE PROPAGATION Introduction Electromagnetic Wave(Radio Waves) travel with a vel. of light. These waves comprises of both Electric and Magnetic Field. The two fields are at right-angles to each other and the direction of propagation is at right-angles to both fields. The Plane of the Electric Field defines the Polarisation of the wave. Field Strength Relationship Electric Field, E x y Magnetic Direction of Field, H z Propagation Electromagnetic Wave(Radio Waves) travel with a vel. of light. These waves comprises of both Electric and Magnetic Field. The two fields are at right-angles to each other and the direction of propagation is at right-angles to both fields. The Plane of the Electric Field defines the Polarisation of the wave. POLARIZATION The polarization of an antenna is the orientation of the electric field with respect to the Earth's surface. Polarization of e.m. Wave is determined by the physical structure of the antenna and by its orientation. Radio waves from a vertical antenna will usually be vertically polarized. Radio waves from a horizontal antenna are usually horizontally polarized. Classification of Radio Wave Propagation GROUND WAVE, SPACE WAVE, SKY WAVE Ground Waves or Surface Waves Ground Waves or Surface Waves ◦ Frequencies up to 2 MHz ◦ follows the curvature of the earth and can travel at distances beyond the horizon (upto some km) ◦ must have vertically polarized antennas ◦ strongest at the low- and medium-frequency ranges ◦ AM broadcast signals are propagated primarily by ground waves during the day and by sky waves at nightis a Surface Wave that propagates or travels close to the surface of the Earth. -
University of Cincinnati
UNIVERSITY OF CINCINNATI _____________ , 20 _____ I,______________________________________________, hereby submit this as part of the requirements for the degree of: ________________________________________________ in: ________________________________________________ It is entitled: ________________________________________________ ________________________________________________ ________________________________________________ ________________________________________________ Approved by: ________________________ ________________________ ________________________ ________________________ ________________________ Digital Direction Finding System Design and Analysis A thesis submitted to the Division of Graduate Studies and Research of the University of Cincinnati in partial fulfillment of the requirements for the degree of MASTER OF SCIENCE (M.S.) in the Department of Electrical & Computer Engineering and Computer Science of the College of Engineering 2003 by Huazhou Liu B.E., Xi’an Jiaotong University P. R. China, 2000 Committee Chair: Professor Howard Fan ABSTRACT Direction Finding (DF) system is used in many military and civilian operations such as surveillance, reconnaissance, and rescue, etc. In the past years, direction finding system is implemented usually using analog RF techniques such as Butler matrix and analog beamforming. Analog direction finding systems have drawbacks inherent from their analog properties such as expensive implementation, inflexibility to adjust or change functionality, intensive calibration procedures and -
Interpretation and Utilization of the Echo
PROCEEDINGS OF THE IEEE, VOL. 62, NO. 6, JUNE 1974 673 Sea Backscatter at HF: Interpretation and Utilization of the Echo DONALD E. BARRICK, MEMBER, IEEE, JAMES M. HEADRICK, SENIOR MEMBER, IEEE, ROBERT W. BOGLE, DOUGLASS D. CROMBIE AND Abstract-Theories and concepts for utilization of HF sea echo are compared and tested against surface-wave measurements made from San Clemente Island in the Pacific in a joint NRL/ITS/NOAA Although the heights of ocean waves are generally small experiment. The use of first-order sea echo as a reference target for in terms of these radar wavelengths, the scattered echo is calibration of HF over-the-horizon radars is established. Features of the higher order Doppler spectrum can be employed to deduce the nonetheless surprisingly large and readily interpretable in principal parameters of the wave-height directional. spectrum (i.e., terms of its Doppler features. The fact that these heights are sea state); and it is shown that significant wave height can be read small facilitates the analysis of scatter using the perturbation from the spectral records. Finally, it is shown that surface currents approximation. This theory [2] produces an equation which and current (depth) gradients can be inferred from the same Doppler 1) agrees with the scattering mechanism deduced by Crombie sea-echo records. from experimental data; 2) properly predicts the positions of I. INTRODUCTION the dominant Doppler peaks; 3) shows how the dominant echo magnitude is related to the sea wave height; and 4) per mits an explanation of some of the less dominant, more com T WENTY YEARS ago Crombie [1] observed sea echo plex features of the sea echo through retention and use of the with an HF radar, and he correctly deduced the scatter higher order terms in the perturbation analysis. -
MUNIS Manual
MUNIS Guide Developed by Computer Education Support in Collaboration with Financial Services Updated February 2019 Table of Contents Logging on to MUNIS ............................................................................ 4 Menus ................................................................................................. 5 Logging Off .......................................................................................... 6 Accounting Code................................................................................... 8 Account Inquiry .................................................................................. 10 Printing a Single Account ..................................................................... 19 Printing a List of Accounts – SBDM Report ............................................. 20 Accounting Code - Quick Review ........................................................... 21 Budget Transfers and Amendments ...................................................... 22 Purchasing and Receiving .................................................................... 29 Model Procurement ............................................................................. 30 Commodity Codes .............................................................................. 33 Vendor Inquiry ................................................................................... 36 Adding an Attachment to Your Requisitions ............................................ 36 Finding Commodity Codes for Warehouse Items......................................37