High Performance, Low Cost and Low Consumption Radio Over Fiber Systems for Diversified Communications Applications
Total Page:16
File Type:pdf, Size:1020Kb
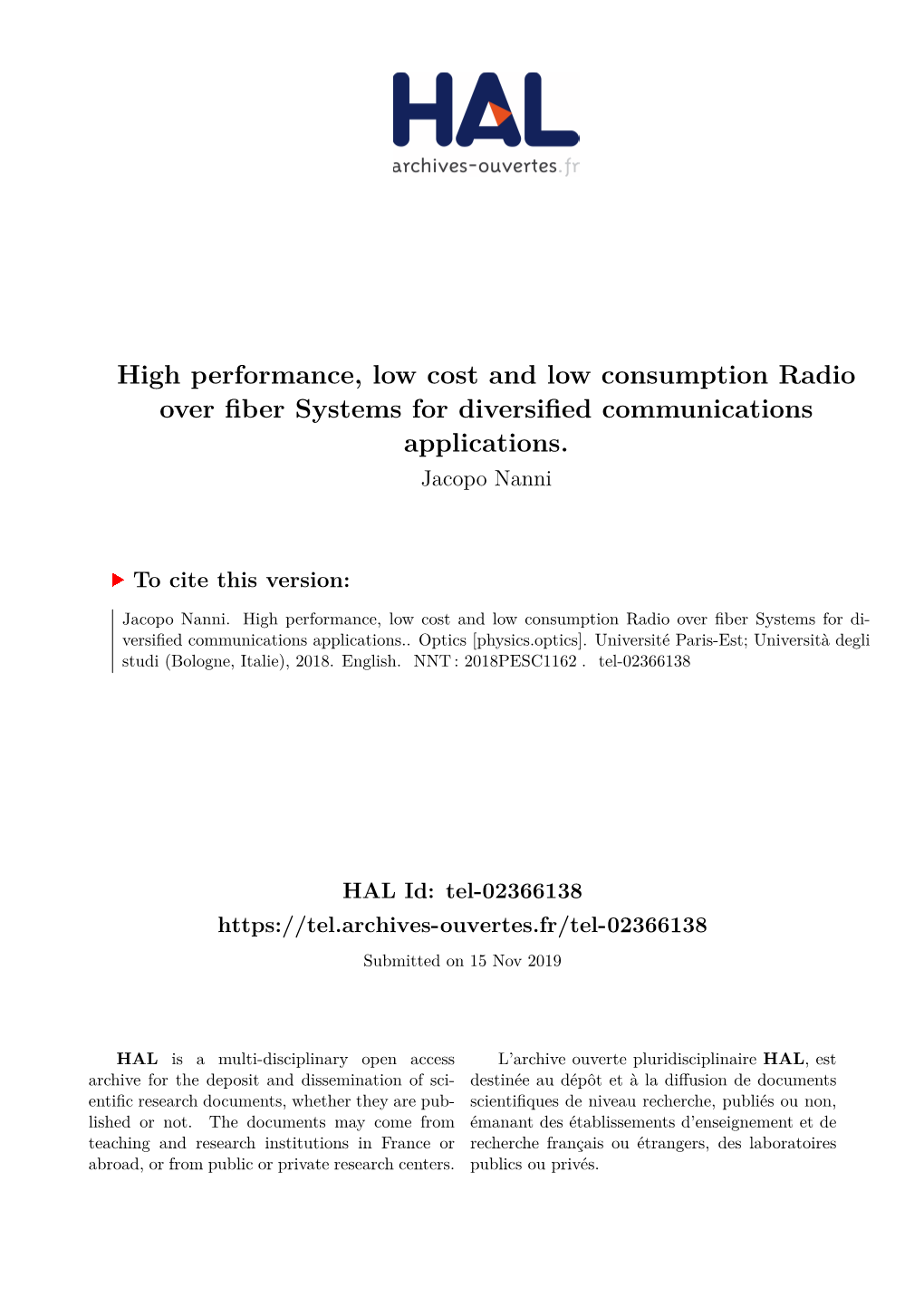
Load more
Recommended publications
-
Radio Over Fiber Communication System: Lateral Shift in Cellular Communication Shagun Singh 1, Suresh Kumar 2 and Payal 3 1M
et International Journal on Emerging Technologies 11 (2): 731-734(2020) ISSN No. (Print): 0975-8364 ISSN No. (Online): 2249-3255 Radio over Fiber Communication System: Lateral Shift in Cellular Communication Shagun Singh 1, Suresh Kumar 2 and Payal 3 1M. Tech. Student, Department of Electronics and Communication Engineering, UIET, MDU Rohtak, (Haryana), India. 2Assistant Professor, Department of Electronics and Communication Engineering, UIET, MDU Rohtak, (Haryana), India. 3Research Scholar, Department of Electronics and Communication Engineering, UIET, MDU Rohtak, (Haryana), India. (Corresponding author: Shagun Singh) (Received 19 December 2019, Revised 26 February 2020, Accepted 28 February 2020) (Published by Research Trend, Website: www.researchtrend.net) ABSTRACT: Cellular technology has progressed with a fast pace. Rolling out of cellular networks upto 4G has already been completed across the world. Research works are going on for finalizing the 5G and 6G technologies. In order to provide secure and high-speed data, Radio over Fiber (RoF) technology has been identified as one of the best means to ensure cost effective and reliable communication facilities. The major challenges is to identify correct modulation techniques, data conversion and link design with suitable components to yield optimum and cost effective solution. In this research paper, we have presented a detailed review of RoF technology, its architectures, latest updates in the field, application in various generations of cellular communication and various modulation techniques. The research gaps exist in the RoF technology have also been highlighted. This will motivate the researchers working in this field of RoF technology to undertake research to bridge the existing gaps. So that, an updated and novel RoF system could be developed and rolled out by the service providers and hard ware manufacturers for the application in cellular technology. -
High Capacity Radio Over Fiber Transmission Links
Downloaded from orbit.dtu.dk on: Oct 05, 2021 High Capacity Radio over Fiber Transmission Links Caballero Jambrina, Antonio Publication date: 2011 Document Version Publisher's PDF, also known as Version of record Link back to DTU Orbit Citation (APA): Caballero Jambrina, A. (2011). High Capacity Radio over Fiber Transmission Links. Technical University of Denmark. General rights Copyright and moral rights for the publications made accessible in the public portal are retained by the authors and/or other copyright owners and it is a condition of accessing publications that users recognise and abide by the legal requirements associated with these rights. Users may download and print one copy of any publication from the public portal for the purpose of private study or research. You may not further distribute the material or use it for any profit-making activity or commercial gain You may freely distribute the URL identifying the publication in the public portal If you believe that this document breaches copyright please contact us providing details, and we will remove access to the work immediately and investigate your claim. i i \main_ACAJ" | 2011/10/17 | 11:46 | page i | #1 i i High Capacity Radio over Fiber Transmission Links Antonio Caballero Jambrina Supervisors: Professor Idelfonso Tafur Monroy, Assistant Professor Darko Zibar and Associate Professor Kresten Yvind Delivery Date: 1st August 2011 DTU Fotonik Department of Photonics Engineering Technical University of Denmark Building 343 2800 Kgs. Lyngby DENMARK i i i i i i \main_ACAJ" | 2011/10/17 | 11:46 | page ii | #2 i i i i i i i i \main_ACAJ" | 2011/10/17 | 11:46 | page i | #3 i i Abstract This thesis expands the state-of-the-art on the detection of high speed wireless signals using optics. -
Radio Over Fiber Technology Tahira Javed, Fatima Amin
International Journal Of Scientific & Engineering Research, Volume 7, Issue 7, July-2016 377 ISSN 2229-5518 Radio over Fiber Technology Tahira Javed, Fatima Amin Abstract— Radio-over-fiber (RoF) is an integration of microwave and optical networks for wireless access. It is a promising technology competent to provide simple antenna front ends, greater wireless access coverage and capacity. RoF system has been dependant on well established technology for base band signals, based on direct detection and intensity modulated links, enable to provide implementation of very low cost. This baseband optical communication with digital signals processing allows advanced modulation formats to be used. The ample advantages of using an optical fiber such as its light weight, less loss, larger bandwidth, tiny size and low cost of cable makes it most suitable for transporting radio signals to antenna sites efficiently, situated remotely in a wireless network. Index Terms— Centralized station, Fiber optic links, IF-over-Fiber, Low-attenuation, Microwave signals, Optical frequency Multiplexing, RF-over-fiber, Radio Frequency amplification, Sub-carrier Multiplexing, Wavelength Division Multiplexing. —————————— —————————— 1 INTRODUCTION The network structure of RoF consists of a Centralized station The Lower power consumption, lower power radiated elec- (CS) which performs modulation, demodulation, medium tromagnetic wave, fibre low attenuation and less loss reduce access control (MAC) coding, routing and other processing’s. the maintenance cost. Due to the simple structure of BS, it is The Centralized Station is connected to several BSs through a highly reliable and system maintenance has become simple. fibre optic. Coverage can be provided by Radio over fibre systems in The most important task of BS is the transformation of optical those areas where direct reception is less effective, like huge signal into wireless signals and vice versa [2]. -
Radio Over Fiber: an Alternative Broadband Network Technology for Iot
electronics Review Radio over Fiber: An Alternative Broadband Network Technology for IoT Diego F. Paredes-Páliz 1,* , Guillermo Royo 1, Francisco Aznar 2, Concepción Aldea 1 and Santiago Celma 1 1 Electronic Design Group—Aragón Institute of Engineering Research, Universidad de Zaragoza, 50009 Zaragoza, Spain; [email protected] (G.R.); [email protected] (C.A.); [email protected] (S.C.) 2 Electronic Design Group—Aragón Institute of Engineering Research, Centro Universitario de la Defensa, 50090 Zaragoza, Spain; [email protected] * Correspondence: [email protected] Received: 22 September 2020; Accepted: 23 October 2020; Published: 27 October 2020 Abstract: Wireless broadband access networks have been positioning themselves as a good solution for manufacturers and users of IoT (internet of things) devices, due mainly to the high data transfer rate required over terminal devices without restriction of information format. In this work, a review of two Radio over Fiber strategies is presented. Both have excellent performance and even offer the possibility to extend wireless area coverage where mobile networks do not reach or the 802.11 network presents issues. Radio Frequency over Fiber (RFoF) and intermediate Frequency over Fiber (IFoF) are two transmission strategies compatible with the required new broadband services and both play a key role in the design of the next generation integrated optical–wireless networks, such as 5G and Satcom networks, including on RAU (Remote Antenna Unit) new functionalities to improve their physical dimensions, employing a microelectronic layout over nanometric technologies. Keywords: IEEE 802.11; intermediate frequency over fiber; internet of things; radio over fiber; wireless access networks 1. Introduction The upcoming internet of things (IoT) technological frontier arouses enormous interest. -
A Review on MIMO Wireless Signals Over Fibre for Next Generation Fibre Wireless (Fiwi) Broadband Networks
electronics Review A Review on MIMO Wireless Signals over Fibre for Next Generation Fibre Wireless (FiWi) Broadband Networks M. A. Elmagzoub , Asadullah Shaikh * , Abdullah Alghamdi and Khairan Rajab College of Computer Science and Information Systems, Najran University, Najran 61441, Saudi Arabia; [email protected] (M.A.E.); [email protected] (A.A.); [email protected] (K.R.) * Correspondence: [email protected] or [email protected] Received: 2 October 2020; Accepted: 24 November 2020; Published: 28 November 2020 Abstract: Next-generation access/mobile networks have set high standards in terms of providing wireless services at high data rates in order to keep up with the vast demands for other mobility and multiple services. Wireless-optical broadband access network (WOBAN) technology, also known as fibre-wireless (FiWi), has uncovered incredible opportunities for the future of next-generation networks because it gets the best of both domains: huge bandwidth provided by the optical fibre and high ubiquity of the wireless domain. The objective of FiWi networks is to integrate the high data rate and long reach provided by optical networks and the ubiquity and mobility of wireless networks, with the target to decrease their expense and complexity. Multiple-input–multiple-output (MIMO) is an inevitable technique for most of the new mobile/wireless networks that are driven by the huge data rates required by today’s users. Consequently, to construct any FiWi system for next-generation (NG) access/broadband networks, an MIMO technique has to be considered. This article presents a comprehensive, contemporary review of the latest subsystems, architectures and integrated technologies of MIMO wireless signals backhauling using optical fibre or fibre access networks, such as passive optical networks (PONs). -
Massively Distributed Antenna Systems with Non-Ideal Optical Front-Hauls
1 Massively Distributed Antenna Systems with Non-Ideal Optical Fiber Front-hauls: A Promising Technology for 6G Wireless Communication Systems Lisu Yu, Member, IEEE, Jingxian Wu, Senior Member, IEEE, Andong Zhou, Erik G. Larsson, Fellow, IEEE, and Pingzhi Fan, Fellow, IEEE Abstract Employing massively distributed antennas brings radio access points (RAPs) closer to users, thus enables aggressive spectrum reuse that can bridge gaps between the scarce spectrum resource and extremely high connection densities in future wireless systems. Examples include cloud radio access network (C-RAN), ultra-dense network (UDN), and cell-free massive multiple-input multiple-output (MIMO) systems. These systems are usually designed in the form of fiber-wireless communications (FWC), where distributed antennas or RAPs are connected to a central unit (CU) through optical front- hauls. A large number of densely deployed antennas or RAPs requires an extensive infrastructure of optical front-hauls. Consequently, the cost, complexity, and power consumption of the network of optical front-hauls may dominate the performance of the entire system. This article provides an overview and outlook on the architecture, modeling, design, and performance of massively distributed antenna systems arXiv:2008.07745v1 [eess.SP] 18 Aug 2020 with non-ideal optical front-hauls. Complex interactions between optical front-hauls and wireless access links require optimum designs across the optical and wireless domains by jointly exploiting their unique L. Yu is with the School of Information Engineering, Nanchang University, Nanchang 330031, P. R. China (e-mail: [email protected]). J. Wu and A. Zhou are with the Department of Electrical Engineering, University of Arkansas, Fayetteville, AR 72701 USA (e-mails: [email protected], [email protected]). -
Radio-Over-Fiber Technologies for Emerging Wireless Systems
> REPLACE THIS LINE WITH YOUR PAPER IDENTIFICATION NUMBER (DOUBLE-CLICK HERE TO EDIT) < 1 Radio-over-Fiber Technologies for Emerging Wireless Systems Dalma Novak, Fellow, IEEE, Rodney B. Waterhouse, Fellow, IEEE, Ampalavanapillai Nirmalathas, Senior Member, IEEE, Christina Lim, Senior Member, IEEE, Prasanna A. Gamage, Member, IEEE, Thomas R. Clark, Jr., Senior Member, IEEE, Michael L. Dennis, Senior Member, IEEE, and Jeffrey A. Nanzer, Senior Member, IEEE use of optical fiber feeder links to extend wireless coverage in Abstract— Radio-over-fiber transmission has been studied radio communication systems [8 – 10]. Since then, the extensively as a means to realizing a fiber optic wireless convergence of optical and wireless networks has continued to distribution network that enables seamless integration of the evolve. Fiber-optic remoting of radio signals is used in a optical and wireless network infrastructures. Emerging wireless diversity of wireless networks, including indoor/in-building communication networks that support new broadband services provide increased opportunities for photonics technologies to distributed antenna systems and outdoor cellular networks. play a prominent role in the realization of next generation The benefits of creating end-to-end integrated network integrated optical/wireless networks. In this paper, we present a solutions that can provide reliable service for both fixed and review of recent developments in radio-over-fiber technologies mobile users, have become well established. that can support the distribution of broadband wireless signals in Today the capabilities of wireless networks are progressing a converged optical/wireless network. We also describe some of more rapidly than ever before. The proliferation of connected the challenges for the successful application of radio-over-fiber technologies in future wireless systems, such as 5G and 60 GHz high capacity smart devices as well as the increase in the networks. -
Survey: Radio Over Fiber and Wireless Broadband Access Technologies
International Journal of Grid and Distributed Computing Vol. 9, No. 3 (2016), pp.83-90 http://dx.doi.org/10.14257/ijgdc.2016.9.3.10 Survey: Radio over Fiber and Wireless Broadband Access Technologies Er. Ravneet kaur1, Er. Harmandar kaur2 1Student at G.N.D.U 2Assistant professor at G.N.D.U [email protected], [email protected] Abstract This paper presents a survey on Radio over fiber for Wireless Broadband Access technology like WiMAX (Fixed and Mobile). Radio over Fiber has achieved an effective delivery of wireless and baseband signal, and has also reduces the power consumption. Whereas recent fast growing broadband access technology through wireless is WiMAX (Worldwide Interoperability for Microwave Access). In this survey, the Radio over fiber implements into wireless broadband access technologies like WiMAX. And also we will be carried out different keying and technical concept from the different methodology. 1. Introduction Network connectivity is categorized either as a backhaul or an access network. In the access network it provides last mile connectivity that connects the end users to the backhaul network which finally connect to the cloud. The recent wireless access network needs to increase coverage range and high data transmission of video, voice and multimedia services with the mobile and fixed users. Therefore for this reason, at the backbone of broadband access technology like WiMAX Base Station (BS), the recent network providers require high transmission bandwidth. So the RoF technology determines a key solution for satisfying this requirement. Since in the backbone wireless and fiber optic technologies, the RoF technology had high bandwidth optical system particularly. -
Transmission System Link for Radio Over Fiber and Wide Area Optical Network 1M.Sivaranjini, 2V.Kasthuri
International Journal of Scientific & Engineering Research Volume 9, Issue 4, April-2018 ISSN 2229-5518 73 Transmission System Link for Radio over Fiber and Wide Area Optical Network 1 2 M.Sivaranjini, V.Kasthuri Abstract— Radio over fiber refers to a transmitted over an optical fiber link. Lower application losses and reduced quality to noise and electromagnetic interference electrical signal transmission. Range of mobile radio signals (3G, 4G, 5G, and WIFI) television signals (ca TV) to the transmission .A wide area network (WAN) is a telecommunication networks or computer network that extends over large geographical distance. ROF system that is modulated with multiple bit rates using different transmission techniques such as stilton and maximum time division multiplexing (MTDW)technique .fiber –radio network comprised two distinct domains, one optical and one wireless. Energy-saving mode, network capacity is dimensional to support peak-hour traffic and IP routers and switches. Network element of during off-peak hours, ideally 75% of energy can be saved the traffic load decreases to its minimal value around 6:00.interconnected network for radio over virtual connection. Keywords— mobile radio signals, over large geographical distance, solution, energy saving mode network, traffic IP and routers, radio signals (3G, 4G, 5G, and Wi-Fi, and television signals. —————————— —————————— addressed. Drawback fiber radio network is a hybrid NTRODUCTION 1 I network that uses a optical network to deliver wireless data Radio networks current network have no energy –saving from in external network. It is Simple and cost effective BSs schema.[1] large amount of energy is wasted when traffic wireless system. -
Low-Cost MIMO Radio Over Fiber System for Multi-Service DAS Using Double Sideband Frequency Translation
View metadata, citation and similar papers at core.ac.uk brought to you by CORE > REPLACE THIS LINE WITH YOUR PAPER IDENTIFICATION NUMBER (DOUBLE-CLICK HERE TO EDIT) < provided1 by Apollo Low-Cost MIMO Radio Over Fiber System for Multi-Service DAS Using Double Sideband Frequency Translation Yumeng Yang, Student Member, IEEE, Michael J. Crisp, Member, IEEE, Richard V. Penty, Senior Member, IEEE and Ian H. White, Fellow, IEEE Abstract—In this paper, a novel low-cost DSB frequency (LTE) and LTE-advanced, the use of MIMO techniques can translation system is experimentally demonstrated and its theory increase data throughput and improve transmission reliability is mathematically proved. The new system is capable of without increasing occupied spectrum. This is particularly transmitting wideband LTE MIMO signals and supporting multiple services. Experimentally, 2×2 LTE MIMO channels with important in indoor environments, where 80-90% of wireless 20MHz bandwidth, and a 700MHz carrier frequency, are data traffic originates [3], but propagation conditions are transmitted simultaneously along with an IEEE 802.11g signal usually poor with a high degree of multipath. (54Mbps) over a 300m length of multi-mode fiber (MMF). The The DAS is one of the most popular methods to increase MIMO channel matrix of the system is retrieved and the condition indoor wireless signal coverage. Many indoor DAS systems use number is calculated. It can be seen in the experimental results RoF technology to transmit signals between a base station (BS) that the system is well conditioned, has a low error vector magnitude (EVM) and the transmission of the MIMO signals has and remote antenna units (RAUs), for versatility, flexibility, negligible effect on the IEEE 802.11g signal. -
Low-Cost MIMO Radio Over Fiber System for Multi-Service DAS Using Double Sideband Frequency Translation
> REPLACE THIS LINE WITH YOUR PAPER IDENTIFICATION NUMBER (DOUBLE-CLICK HERE TO EDIT) < 1 Low-Cost MIMO Radio Over Fiber System for Multi-Service DAS Using Double Sideband Frequency Translation Yumeng Yang, Student Member, IEEE, Michael J. Crisp, Member, IEEE, Richard V. Penty, Senior Member, IEEE and Ian H. White, Fellow, IEEE Abstract—In this paper, a novel low-cost DSB frequency (LTE) and LTE-advanced, the use of MIMO techniques can translation system is experimentally demonstrated and its theory increase data throughput and improve transmission reliability is mathematically proved. The new system is capable of without increasing occupied spectrum. This is particularly transmitting wideband LTE MIMO signals and supporting multiple services. Experimentally, 2×2 LTE MIMO channels with important in indoor environments, where 80-90% of wireless 20MHz bandwidth, and a 700MHz carrier frequency, are data traffic originates [3], but propagation conditions are transmitted simultaneously along with an IEEE 802.11g signal usually poor with a high degree of multipath. (54Mbps) over a 300m length of multi-mode fiber (MMF). The The DAS is one of the most popular methods to increase MIMO channel matrix of the system is retrieved and the condition indoor wireless signal coverage. Many indoor DAS systems use number is calculated. It can be seen in the experimental results RoF technology to transmit signals between a base station (BS) that the system is well conditioned, has a low error vector magnitude (EVM) and the transmission of the MIMO signals has and remote antenna units (RAUs), for versatility, flexibility, negligible effect on the IEEE 802.11g signal. -
Space Division Multiplexing 5G Fronthaul with Analog and Digital Radio-Over-Fiber and Optical Beamforming – the Bluespace Concept
Space Division Multiplexing 5G Fronthaul with Analog and Digital Radio-over-Fiber and Optical Beamforming – the blueSPACE Concept Dissemination level Public Publication date 24.08.2018 Publisher Eindhoven University of Technology DOI 10.5281/zenodo.1403140 blueSPACE is a phase 2 project of the 5G Infrastructure Public Private Partnership, 5G-PPP. This project has received funding from the European Union’s Horizon 2020 research and innovation programme under grant agreement No 762055. 762055— blueSPACE — H2020-ICT-2016-2 Document information Authors Idelfonso Tafur Monroy, Simon Rommel – TU/e Josep M. Fabrega, Raul Munoz – CTTC Maria Carmen Vazquez Garcia, David Larrabeiti – UC3M Salvador Sales, Diego Perez Galacho – UPV Jaroslaw P. Turkiewicz, Emil Kowalczyk – OP Dimitrios Klonidis, Ioannis Tomkos – AIT Gilles Feugnet, Jerome Bourderionnet – THALES Evangelos Grivas, Thomas Nikas, Dimitrios Syvridis – EULAMBIA Chris Roeloffzen, Jörn Epping, Paul van Dijk – LioniX Dimitrios Kritharidis, Konstantinos Chartsias – INTRACOM Giada Landi, Marco Capitani – NXW Michael Eiselt – ADVA Mykhaylo Dubov – OPT Editor Simon Rommel – TU/e Project funding 762055— blueSPACE — H2020-ICT-2016-2 Short summary This paper presents the concept of the blueSPACE project. The blueSPACE project is one of the 21 second phase 5G-PPP initiative European H2020 projects focusing on delivering solutions, architectures, technologies and standards for the ubiquitous next generation communication infrastructures. This concept paper presents the blueSPACE proposition for the use of spatial division multiplexing for 5G fronthauling, the use of optical beamforming from Ka-band wireless signals, the incorporation of power-over-fiber distribution and the integration into a flexible SDN/NFV environment. Also, the chosen use cases and a global description of the planned demonstrations are presented.