Owen DW Phd 2015.Pdf
Total Page:16
File Type:pdf, Size:1020Kb
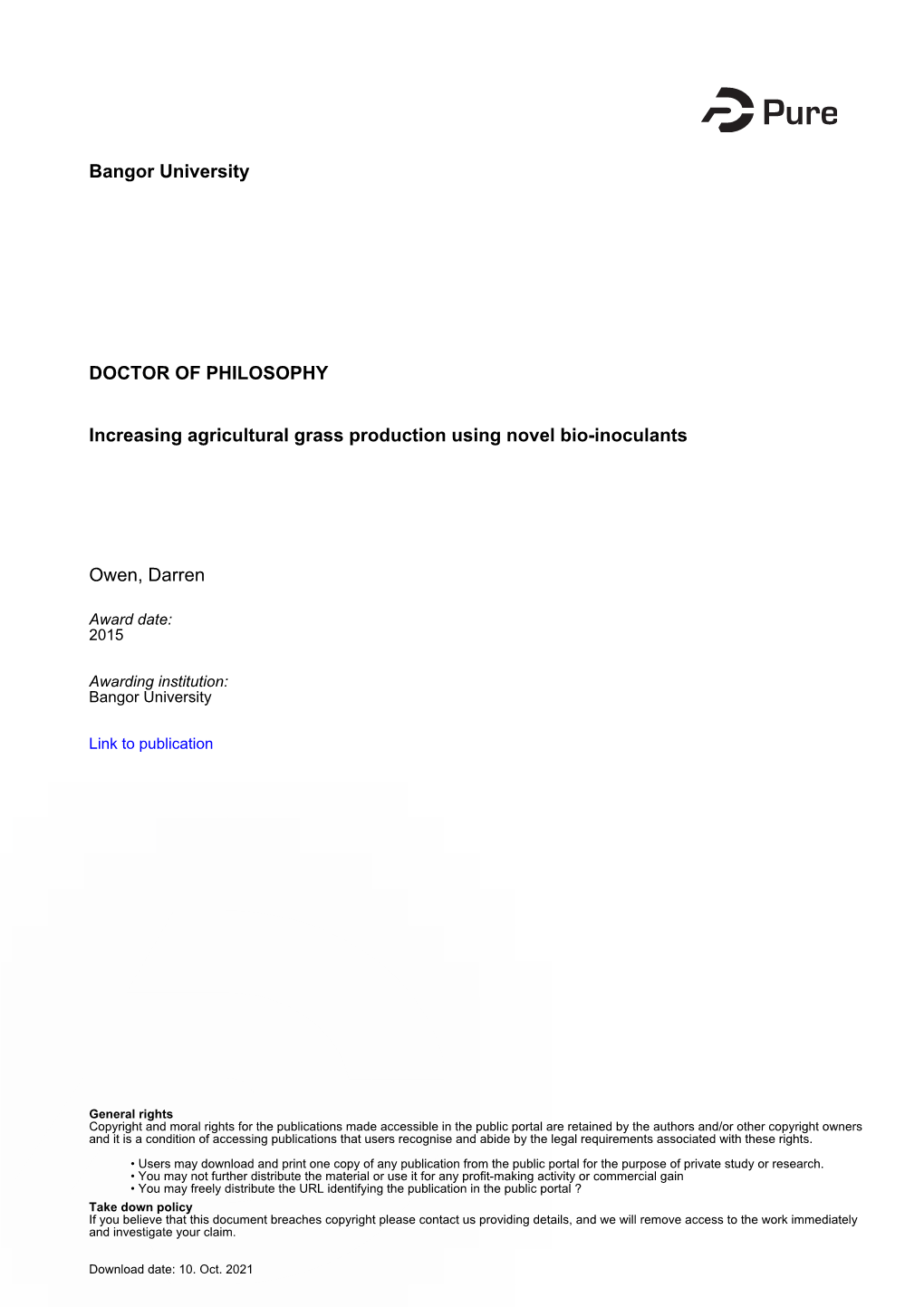
Load more
Recommended publications
-
<I>Serendipita Sacchari</I>
MYCOTAXON ISSN (print) 0093-4666 (online) 2154-8889 Mycotaxon, Ltd. ©2020 July–September 2020—Volume 135, pp. 579–587 https://doi.org/10.5248/135.579 Serendipita sacchari sp. nov. from a sugarcane rhizosphere in southern China Ling Xie1,2#, Yan-Yan Long1,2#, Yan Zhang1,2, Yan-Lu Chen1,2, Wen-Long Zhang2* 1Plant Protection Research Institute, Guangxi Academy of Agricultural Science, Nanning 530007, China 2 Microbiology Research Institute, Guangxi Academy of Agricultural Science, Nanning 530007, China *Correspondence to: [email protected] Abstract—We isolated a new species, proposed here as Serendipita sacchari, from a sugarcane rhizosphere in Guangxi Province, China. This species is characterized by its unstable nucleus numbers (1–15) in its chlamydospores versus their regular distribution in hyphal cells. ITS rDNA and combined LSU+ TEF1-α sequence analyses also support the uniqueness of this new plant symbiont. Key words—molecular phylogeny, Sebacinales, Serendipitaceae, taxonomy Introduction Serendipita P. Roberts (Basidiomycota, Sebacinales, Serendipitaceae), typified with S. vermifera (Oberw.) P. Roberts, originally comprised seven species (Roberts 1993). Two additional new species S. lyrica Trichiès (Trichiès 2003), and S. herbamans K. Riess & al. (Riess & al. 2014) have been proposed in this genus, and two anamorphic species in Piriformospora Sav. Verma & al. have been recombined as S. indica (Sav. Verma & al.) M. Weiss & al., and S. williamsii (Zuccaro & M. Weiss) M. Weiss & al. (Verma & al. 1998; Basiewicz & al. 2012; Weiß & al. 2016). Serendipita currently contains 11 species and DNA barcodes are widely accepted as an important tool in delineating species (Schoch & al. 2012; Riess & al. 2014). # Ling Xie & Yan-Yan Long contributed equally to this work. -
Bodenmikrobiologie (Version: 07/2019)
Langzeitmonitoring von Ökosystemprozessen - Methoden-Handbuch Modul 04: Bodenmikrobiologie (Version: 07/2019) www.hohetauern.at Impressum Impressum Für den Inhalt verantwortlich: Dr. Fernando Fernández Mendoza & Prof. Mag Dr. Martin Grube Institut für Biologie, Bereich Pflanzenwissenschaften, Universität Graz, Holteigasse 6, 8010 Graz Nationalparkrat Hohe Tauern, Kirchplatz 2, 9971 Matrei i.O. Titelbild: Ein Transekt im Untersuchungsgebiet Innergschlöss (2350 m üNN) wird im Jahr 2017 beprobt. © Newesely Zitiervorschlag: Fernández Mendoza F, Grube M (2019) Langzeitmonitoring von Ökosystemprozessen im Nationalpark Hohe Tauern. Modul 04: Mikrobiologie. Methoden-Handbuch. Verlag der Österreichischen Akademie der Wissenschaften, Wien. ISBN-Online: 978-3-7001-8752-3, doi: 10.1553/GCP_LZM_NPHT_Modul04 Weblink: https://verlag.oeaw.ac.at und http://www.parcs.at/npht/mmd_fullentry.php?docu_id=38612 Inhaltsverzeichnis Zielsetzung ...................................................................................................................................................... 1 Inhalt Vorbereitungsarbeit und benötigtes Material ................................................................................................... 2 a. Materialien für die Probenahme und Probenaufbewahrung ................................................................ 2 b. Materialien und Geräte für die Laboranalyse ...................................................................................... 2 Arbeitsablauf ................................................................................................................................................... -
The Flora Mycologica Iberica Project Fungi Occurrence Dataset
A peer-reviewed open-access journal MycoKeys 15: 59–72 (2016)The Flora Mycologica Iberica Project fungi occurrence dataset 59 doi: 10.3897/mycokeys.15.9765 DATA PAPER MycoKeys http://mycokeys.pensoft.net Launched to accelerate biodiversity research The Flora Mycologica Iberica Project fungi occurrence dataset Francisco Pando1, Margarita Dueñas1, Carlos Lado1, María Teresa Telleria1 1 Real Jardín Botánico-CSIC, Claudio Moyano 1, 28014, Madrid, Spain Corresponding author: Francisco Pando ([email protected]) Academic editor: C. Gueidan | Received 5 July 2016 | Accepted 25 August 2016 | Published 13 September 2016 Citation: Pando F, Dueñas M, Lado C, Telleria MT (2016) The Flora Mycologica Iberica Project fungi occurrence dataset. MycoKeys 15: 59–72. doi: 10.3897/mycokeys.15.9765 Resource citation: Pando F, Dueñas M, Lado C, Telleria MT (2016) Flora Mycologica Iberica Project fungi occurrence dataset. v1.18. Real Jardín Botánico (CSIC). Dataset/Occurrence. http://www.gbif.es/ipt/resource?r=floramicologicaiberi ca&v=1.18, http://doi.org/10.15468/sssx1e Abstract The dataset contains detailed distribution information on several fungal groups. The information has been revised, and in many times compiled, by expert mycologist(s) working on the monographs for the Flora Mycologica Iberica Project (FMI). Records comprise both collection and observational data, obtained from a variety of sources including field work, herbaria, and the literature. The dataset contains 59,235 records, of which 21,393 are georeferenced. These correspond to 2,445 species, grouped in 18 classes. The geographical scope of the dataset is Iberian Peninsula (Continental Portugal and Spain, and Andorra) and Balearic Islands. The complete dataset is available in Darwin Core Archive format via the Global Biodi- versity Information Facility (GBIF). -
Jason Stajich UC Riverside Fungidb Supported by Sloan Foundation
FungiDB and 1000 Fungal genomes Jason Stajich UC Riverside FungiDB supported by Sloan Foundation • Data coordinating center - Knight, Sogin, Meyer labs • Fungal microbiome support - Stajich Lab (Greg Gu, Steven Ahrendt) --> Scott Bates, Jon Leff Fierer Lab Fungal genome sequencing * ** 10-15 “Zygos” + Chytrids + Cryptomycota 400-500+ genomes of Fungi http://www.diark.org/diark/statistics http://1000.fungalgenomes.org Addressing the phylogenetic diversity: 1000 Fungal genomes project !"#$%&'%()*+#+,- !"#$%&'%()*+#+,- .%#$'/+%()*+#+,- .%#$'/+%()*+#+,- 01"%2%()*+#+,- 01"%2%()*+#+,- D+%E8%,,%()*+#+,- D+%E8%,,%()*+#+,- F+G'G%()*%2&4- 3&*+"#4+-,+/',- F+G'G%()*%2&4- 3&*+"#4+-,+/',- 547%187+&'%()*+#+,- 547%187+&'%()*+#+,- 5+*4&%"%()*+#+,- 5+*4&%"%()*+#+,- 5+%2%()*+#+,- 5+%2%()*+#+,- 5'*$'&%()*+#+,- 5'*$'&%()*+#+,- 6"7'8'%()*+#+,- 6"7'8'%()*+#+,- F+G'G%()*+#+,- F+G'G%()*+#+,- H4**$4"%()*%2&4- H%"/4"'%()*+#+,- H4**$4"%()*%2&4- H%"/4"'%()*+#+,- H4**$4"%()*+#+,- H4**$4"%()*+#+,- I+%8+*#%()*+#+,- I+%8+*#%()*+#+,- J4K$"'&%()*%2&4- F&+1(%*),2/'%()*+#+,- J4K$"'&%()*%2&4- F&+1(%*),2/'%()*+#+,- H*$'G%,4**$4"%()*+#+,- H*$'G%,4**$4"%()*+#+,- J4K$"'&%()*+#+,- J4K$"'&%()*+#+,- M,284E'&%()*%2&4- 0L%74,'/'%()*+#+,- M,284E'&%()*%2&4- 0L%74,'/'%()*+#+,- M,284E'&%()*+#+,- M,284E'&%()*+#+,- !E4"'*%,287%()*+#+,- !E4"'*%,287%()*+#+,- !#"4*2+88%()*+#+,- !#"4*2+88%()*+#+,- N84,,'*18%()*+#+,- N84,,'*18%()*+#+,- F1**'&'%()*%2&4- N")K#%()*%*%84*%()*+#+,- F1**'&'%()*%2&4- N")K#%()*%*%84*%()*+#+,- N),#%74,'/'%()*+#+,- N),#%74,'/'%()*+#+,- O'*"%7%#")%()*+#+,- O'*"%7%#")%()*+#+,- -
Crittendenia Gen. Nov., a New Lichenicolous Lineage in the Agaricostilbomycetes (Pucciniomycotina), and a Review of the Biology
The Lichenologist (2021), 53, 103–116 doi:10.1017/S002428292000033X Standard Paper Crittendenia gen. nov., a new lichenicolous lineage in the Agaricostilbomycetes (Pucciniomycotina), and a review of the biology, phylogeny and classification of lichenicolous heterobasidiomycetes Ana M. Millanes1, Paul Diederich2, Martin Westberg3 and Mats Wedin4 1Departamento de Biología y Geología, Física y Química Inorgánica, Universidad Rey Juan Carlos, E-28933 Móstoles, Spain; 2Musée national d’histoire naturelle, 25 rue Munster, L-2160 Luxembourg; 3Museum of Evolution, Norbyvägen 16, SE-75236 Uppsala, Sweden and 4Department of Botany, Swedish Museum of Natural History, P.O. Box 50007, SE-10405 Stockholm, Sweden Abstract The lichenicolous ‘heterobasidiomycetes’ belong in the Tremellomycetes (Agaricomycotina) and in the Pucciniomycotina. In this paper, we provide an introduction and review of these lichenicolous taxa, focusing on recent studies and novelties of their classification, phylogeny and evolution. Lichen-inhabiting fungi in the Pucciniomycotina are represented by only a small number of species included in the genera Chionosphaera, Cyphobasidium and Lichenozyma. The phylogenetic position of the lichenicolous representatives of Chionosphaera has, however, never been investigated by molecular methods. Phylogenetic analyses using the nuclear SSU, ITS, and LSU ribosomal DNA mar- kers reveal that the lichenicolous members of Chionosphaera form a monophyletic group in the Pucciniomycotina, distinct from Chionosphaera and outside the Chionosphaeraceae. The new genus Crittendenia is described to accommodate these lichen-inhabiting spe- cies. Crittendenia is characterized by minute synnemata-like basidiomata, the presence of clamp connections and aseptate tubular basidia from which 4–7 spores discharge passively, often in groups. Crittendenia, Cyphobasidium and Lichenozyma are the only lichenicolous lineages known so far in the Pucciniomycotina, whereas Chionosphaera does not include any lichenicolous taxa. -
New Records of Aspergillus Allahabadii and Penicillium Sizovae
MYCOBIOLOGY 2018, VOL. 46, NO. 4, 328–340 https://doi.org/10.1080/12298093.2018.1550169 RESEARCH ARTICLE Four New Records of Ascomycete Species from Korea Thuong T. T. Nguyen, Monmi Pangging, Seo Hee Lee and Hyang Burm Lee Division of Food Technology, Biotechnology and Agrochemistry, College of Agriculture & Life Sciences, Chonnam National University, Gwangju, Korea ABSTRACT ARTICLE HISTORY While evaluating fungal diversity in freshwater, grasshopper feces, and soil collected at Received 3 July 2018 Dokdo Island in Korea, four fungal strains designated CNUFC-DDS14-1, CNUFC-GHD05-1, Revised 27 September 2018 CNUFC-DDS47-1, and CNUFC-NDR5-2 were isolated. Based on combination studies using Accepted 28 October 2018 phylogenies and morphological characteristics, the isolates were confirmed as Ascodesmis KEYWORDS sphaerospora, Chaetomella raphigera, Gibellulopsis nigrescens, and Myrmecridium schulzeri, Ascomycetes; fecal; respectively. This is the first records of these four species from Korea. freshwater; fungal diversity; soil 1. Introduction Paraphoma, Penicillium, Plectosphaerella, and Stemphylium [7–11]. However, comparatively few Fungi represent an integral part of the biomass of any species of fungi have been described [8–10]. natural environment including soils. In soils, they act Freshwater nourishes diverse habitats for fungi, as agents governing soil carbon cycling, plant nutri- such as fallen leaves, plant litter, decaying wood, tion, and pathology. Many fungal species also adapt to aquatic plants and insects, and soils. Little -
9B Taxonomy to Genus
Fungus and Lichen Genera in the NEMF Database Taxonomic hierarchy: phyllum > class (-etes) > order (-ales) > family (-ceae) > genus. Total number of genera in the database: 526 Anamorphic fungi (see p. 4), which are disseminated by propagules not formed from cells where meiosis has occurred, are presently not grouped by class, order, etc. Most propagules can be referred to as "conidia," but some are derived from unspecialized vegetative mycelium. A significant number are correlated with fungal states that produce spores derived from cells where meiosis has, or is assumed to have, occurred. These are, where known, members of the ascomycetes or basidiomycetes. However, in many cases, they are still undescribed, unrecognized or poorly known. (Explanation paraphrased from "Dictionary of the Fungi, 9th Edition.") Principal authority for this taxonomy is the Dictionary of the Fungi and its online database, www.indexfungorum.org. For lichens, see Lecanoromycetes on p. 3. Basidiomycota Aegerita Poria Macrolepiota Grandinia Poronidulus Melanophyllum Agaricomycetes Hyphoderma Postia Amanitaceae Cantharellales Meripilaceae Pycnoporellus Amanita Cantharellaceae Abortiporus Skeletocutis Bolbitiaceae Cantharellus Antrodia Trichaptum Agrocybe Craterellus Grifola Tyromyces Bolbitius Clavulinaceae Meripilus Sistotremataceae Conocybe Clavulina Physisporinus Trechispora Hebeloma Hydnaceae Meruliaceae Sparassidaceae Panaeolina Hydnum Climacodon Sparassis Clavariaceae Polyporales Gloeoporus Steccherinaceae Clavaria Albatrellaceae Hyphodermopsis Antrodiella -
Using Rdptools Output with Phyloseq John Quensen & Qiong Wang October 23, 2015
Using RDPTools Output with Phyloseq John Quensen & Qiong Wang October 23, 2015 Introduction The purpose of this document is to demonstrate how to generate RDPTools (Cole et al., 2014) output from sequencing data and import it into the R/Bioconductor package phyloseq (McMurdie and Holmes, 2012). Once this is done, the data can be analyzed not only using phyloseq’s wrapper functions, but by any method available in R. For examples see the section Examples of Data Analysis below. You may install R from the Comprehensive R Archive Network (CRAN) repository at http://cran.us. r-project.org/. As an aid to working with R, we suggest that you also install RStudio, an IDE interface for R, available at http://www.rstudio.com/. By loading the Rmd file included with the tutorial files (see below) into RStudio, you can easily recreate the R portions of these tutorials on your own computer. And you can get an explanation of how to use any function in a code block by placing the cursor anywhere in the function name and pressing key F1. RDPTools The Ribosomal Database Project (RDP) at Michigan State University has long provided web-based tools for processing pyrosequencing data. These tools were originally developed for handling data for bacterial and archaeal 16S rRNA genes, but since then their capabilities have been expanded to include functional genes, 28S rRNA and ITS fungal sequences, and Illumina data. To handle the increased demands of analyzing larger data sets, command line versions of the tools have been made available as RDPTools on GitHub (https://github.com/rdpstaff/RDPTools). -
Crittendenia Gen. Nov., a New Lichenicolous Lineage in the Agaricostilbomycetes (Pucciniomycotina), and a Review of the Biology
The Lichenologist (2021), 53, 103–116 doi:10.1017/S002428292000033X Standard Paper Crittendenia gen. nov., a new lichenicolous lineage in the Agaricostilbomycetes (Pucciniomycotina), and a review of the biology, phylogeny and classification of lichenicolous heterobasidiomycetes Ana M. Millanes1, Paul Diederich2, Martin Westberg3 and Mats Wedin4 1Departamento de Biología y Geología, Física y Química Inorgánica, Universidad Rey Juan Carlos, E-28933 Móstoles, Spain; 2Musée national d’histoire naturelle, 25 rue Munster, L-2160 Luxembourg; 3Museum of Evolution, Norbyvägen 16, SE-75236 Uppsala, Sweden and 4Department of Botany, Swedish Museum of Natural History, P.O. Box 50007, SE-10405 Stockholm, Sweden Abstract The lichenicolous ‘heterobasidiomycetes’ belong in the Tremellomycetes (Agaricomycotina) and in the Pucciniomycotina. In this paper, we provide an introduction and review of these lichenicolous taxa, focusing on recent studies and novelties of their classification, phylogeny and evolution. Lichen-inhabiting fungi in the Pucciniomycotina are represented by only a small number of species included in the genera Chionosphaera, Cyphobasidium and Lichenozyma. The phylogenetic position of the lichenicolous representatives of Chionosphaera has, however, never been investigated by molecular methods. Phylogenetic analyses using the nuclear SSU, ITS, and LSU ribosomal DNA mar- kers reveal that the lichenicolous members of Chionosphaera form a monophyletic group in the Pucciniomycotina, distinct from Chionosphaera and outside the Chionosphaeraceae. The new genus Crittendenia is described to accommodate these lichen-inhabiting spe- cies. Crittendenia is characterized by minute synnemata-like basidiomata, the presence of clamp connections and aseptate tubular basidia from which 4–7 spores discharge passively, often in groups. Crittendenia, Cyphobasidium and Lichenozyma are the only lichenicolous lineages known so far in the Pucciniomycotina, whereas Chionosphaera does not include any lichenicolous taxa. -
Pilzgattungen Europas
Pilzgattungen Europas - Liste 3: Notizbuchartige Auswahlliste zur Bestimmungsliteratur für Aphyllophorales und Heterobasidiomyceten (ohne cyphelloide Pilze und ohne Rost- und Brandpilze) Bernhard Oertel INRES Universität Bonn Auf dem Hügel 6 D-53121 Bonn E-mail: [email protected] 24.06.2011 Gattungen 1) Hauptliste 2) Liste der heute nicht mehr gebräuchlichen Gattungsnamen (Anhang) 1) Hauptliste Abortiporus Murr. 1904 (muss Loweomyces hier dazugeschlagen werden?): Lebensweise: Z.T. phytoparasitisch an Wurzeln von Bäumen Typus: A. distortus (Schw. : Fr.) Murr. [= Boletus distortus Schw. : Fr.; heute: A. biennis (Bull. : Fr.) Sing.; Anamorfe: Sporotrichopsis terrestris (Schulz.) Stalpers; Synonym der Anamorfe: Ceriomyces terrestris Schulz.] Bestimm. d. Gatt.: Bernicchia (2005), 68 u. 74 (auch Arten- Schlüssel); Bresinsky u. Besl (2003), 64; Hansen u. Knudsen 3 (1997), 220; Jülich (1984), 37-38 u. 328; Pegler (1973), The Fungi 4B, 404; Ryvarden u. Gilbertson (1993), Bd. 1, 70 u. 81 (auch Arten- Schlüssel) Abb.: 2) Lit.: Bollmann, Gminder u. Reil-CD (2007) Fidalgo, O. (1969), Revision ..., Rickia 4, 99-208 Jahn (1963), 65 Lohmeyer, T.R. (2000), Porlinge zwischen Inn und Salzach ..., Mycol. Bavarica 4, 33-47 Moser et al. (1985 ff.), Farbatlas (Gatt.-beschr.) Murrill (1904), Bull. Torrey Bot. Club 31, 421 Ryvarden u. Gilbertson (1993), Bd. 1, 81 s. ferner in 1) Abundisporus Ryv. 1999 [Europa?]: Typus: A. fuscopurpureus (Pers.) Ryv. (= Polyporus fuscopurpureus Pers.) Lit.: Ryvarden, L. ("1998", p. 1999), African polypores ..., Belg. J. Bot. 131 [Heinemann-Festschrift], 150- 155 (S. 154) s. ferner in 1) Acanthobasidium Oberw. 1965 (zu Aleurodiscus?): Typus: A. delicatum (Wakef.) Oberw. ex Jül. (= Aleurodiscus delicatus Wakef.) Bestimm. d. Gatt.: Bernicchia u. -
MMA MASTERLIST - Sorted by Taxonomy
MMA MASTERLIST - Sorted by Taxonomy Sunday, December 10, 2017 Page 1 of 86 Amoebozoa Mycetomycota Protosteliomycetes Protosteliales Ceratiomyxaceae Ceratiomyxa fruticulosa Ceratiomyxa fruticulosa var. fruticulosa Ceratiomyxa fruticulosa var. poroides Ceratiomyxa sp. Mycetozoa Myxogastrea Incertae Sedis in Myxogastrea Liceaceae Licea minima Stemonitidaceae Brefeldia maxima Comatricha pulchella Comatricha sp. Comatricha typhoides Stemonitis axifera Stemonitis fusca Stemonitis sp. Stemonitis splendens Chromista Oomycota Incertae Sedis in Oomycota Peronosporales Peronosporaceae Plasmopara viticola Pythiaceae Pythium deBaryanum Oomycetes Saprolegniales Saprolegniaceae Saprolegnia sp. Peronosporea Albuginales Albuginaceae Albugo candida Fungus Ascomycota Ascomycetes Boliniales Boliniaceae Camarops petersii Capnodiales Capnodiaceae Scorias spongiosa Diaporthales Gnomoniaceae Cryptodiaporthe corni Sydowiellaceae Stegophora ulmea Valsaceae Cryphonectria parasitica Valsella nigroannulata Elaphomycetales Elaphomycetaceae Elaphomyces granulatus Elaphomyces sp. Erysiphales Erysiphaceae Erysiphe aggregata Erysiphe cichoracearum Erysiphe polygoni Microsphaera extensa Phyllactinia guttata Podosphaera clandestina Uncinula adunca Uncinula necator Hysteriales Hysteriaceae Glonium stellatum Leotiales Bulgariaceae Crinula caliciiformis Crinula sp. Mycocaliciales Mycocaliciaceae Phaeocalicium polyporaeum Peltigerales Collemataceae Leptogium cyanescens Lobariaceae Sticta fimbriata Nephromataceae Nephroma helveticum Peltigeraceae Peltigera evansiana Peltigera -
Orbilia Ultrastructure, Character Evolution and Phylogeny of Pezizomycotina
Mycologia, 104(2), 2012, pp. 462–476. DOI: 10.3852/11-213 # 2012 by The Mycological Society of America, Lawrence, KS 66044-8897 Orbilia ultrastructure, character evolution and phylogeny of Pezizomycotina T.K. Arun Kumar1 INTRODUCTION Department of Plant Biology, University of Minnesota, St Paul, Minnesota 55108 Ascomycota is a monophyletic phylum (Lutzoni et al. 2004, James et al. 2006, Spatafora et al. 2006, Hibbett Rosanne Healy et al. 2007) comprising three subphyla, Taphrinomy- Department of Plant Biology, University of Minnesota, cotina, Saccharomycotina and Pezizomycotina (Su- St Paul, Minnesota 55108 giyama et al. 2006, Hibbett et al. 2007). Taphrinomy- Joseph W. Spatafora cotina, according to the current classification (Hibbett Department of Botany and Plant Pathology, Oregon et al. 2007), consists of four classes, Neolectomycetes, State University, Corvallis, Oregon 97331 Pneumocystidiomycetes, Schizosaccharomycetes, Ta- phrinomycetes, and an unplaced genus, Saitoella, Meredith Blackwell whose members are ecologically and morphologically Department of Biological Sciences, Louisiana State University, Baton Rouge, Louisiana 70803 highly diverse (Sugiyama et al. 2006). Soil Clone Group 1, poorly known from geographically wide- David J. McLaughlin spread environmental samples and a single culture, Department of Plant Biology, University of Minnesota, was suggested as a fourth subphylum (Porter et al. St Paul, Minnesota 55108 2008). More recently however the group has been described as a new class of Taphrinomycotina, Archae- orhizomycetes (Rosling et al. 2011), based primarily on Abstract: Molecular phylogenetic analyses indicate information from rRNA sequences. The mode of that the monophyletic classes Orbiliomycetes and sexual reproduction in Taphrinomycotina is ascogen- Pezizomycetes are among the earliest diverging ous without the formation of ascogenous hyphae, and branches of Pezizomycotina, the largest subphylum except for the enigmatic, apothecium-producing of the Ascomycota.