Measurement of Ionospheric Absorption by the Cosmic Radio Noise Method
Total Page:16
File Type:pdf, Size:1020Kb
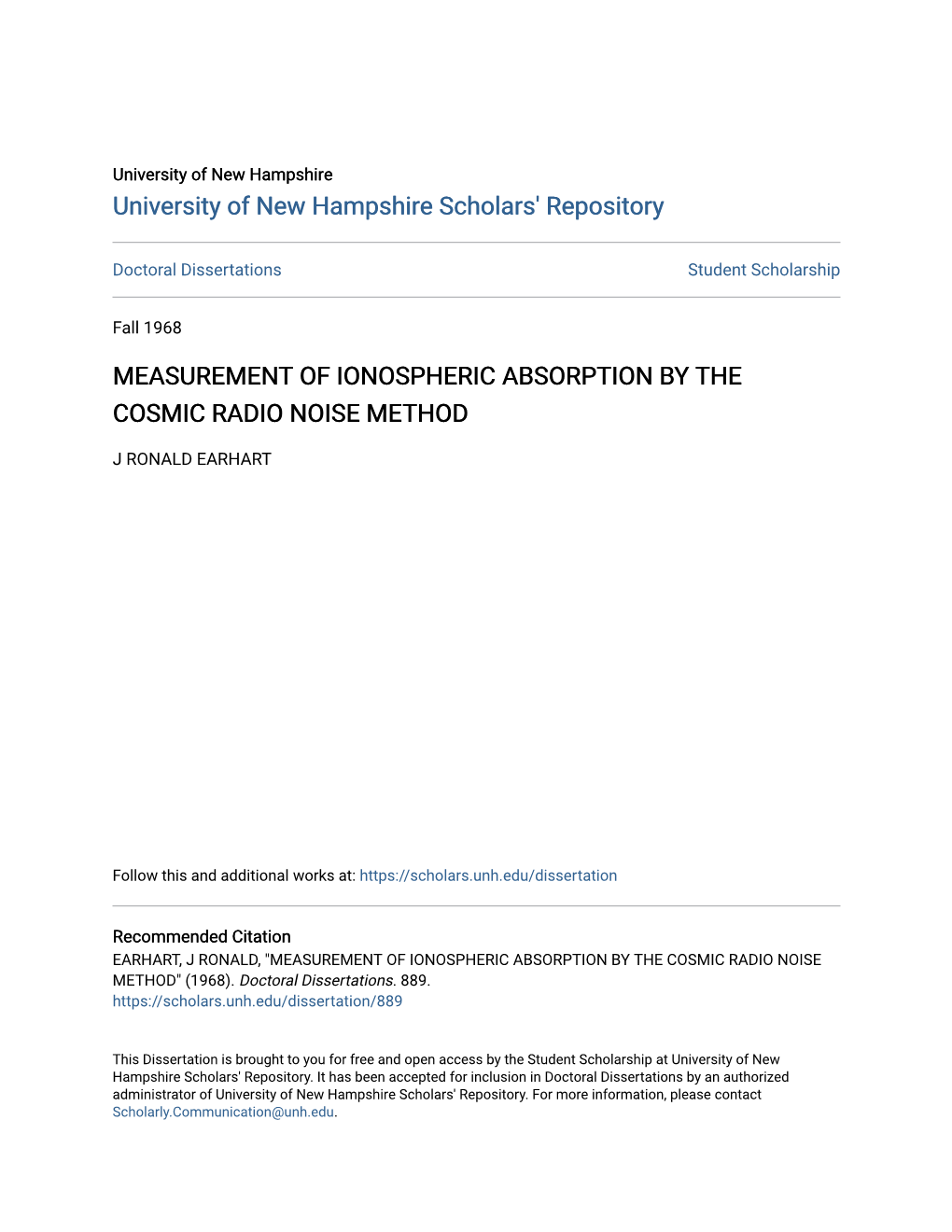
Load more
Recommended publications
-
Spectrum Management Technical Handbook
NTIA TN 89-2 SPECTRUM MANAGEMENT TECHNICAL HANDBOOK TECHNICAL NOTE SERIES NTIA TECHNICAL NOTE 89-2 SPECTRUM MANAGEMENT TECHNICAL HANDBOOK l U.S. DEPARTMENT OF COMMERCE Robert A. Mosbacher, Secretary Alfred C. Sikes, Assistant Secretary for Communications and Information JULY 1989 ACKNOWLEDGEMENT NT IA acknowledges the efforts of Mark Schellhammer in the completion of Phase I of this handbook (Sections 1-3). Other portions will be added as resources are available. ABSTRACT This handbook is a compilation of the procedures, definitions, relationships and conversions essential to electromagnetic compatibility analysis. It is intended as a reference for NTIA engineers. KEY WORDS CONVERSION FACTORS COUPLING EQUATIONS DEFINITIONS ·TECHNICAL RELATIONSHIPS iii TABLE OF CONTENTS Subsection Page SECTION 1 TECHNICAL TERMS:DEFINITIONS AND NOTATION TERMS AND DEFINITIONS . .. .. 1-1 GLOSSARY OF STANDARD NOTATION . .. 1-13 SECTION 2 TECHNICAL RELATIONSHIPS AND CONVERSION FACTORS INTRODUCTION . .. .. .. 2-1 TRANSMISSION LOSS FOR RADIO LINKS . �. .. .. 2-1 Propagation Loss . .. .. .. 2-1 Transmission Loss ........................................ 2-2 Free-Space Basic Transmission Loss .......................... 2-2 Basic Transmission Loss .................................... 2-2 Loss Relative to Free-Space .................................. 2-2 System Loss . .. 2-3 ; Total Loss . .. .. 2-3 ANTENNA CHARACTERISTICS . .. 2-3 Antenna Directivity ........................................ 2-3 Antenna Gain and Efficiency .................................. 2-3 I -
A Novel Ship Target Detection Algorithm Based on Error Self-Adjustment Extreme Learning Machine and Cascade Classifier
Cognitive Computation (2019) 11:110–124 https://doi.org/10.1007/s12559-018-9606-5 A Novel Ship Target Detection Algorithm Based on Error Self-adjustment Extreme Learning Machine and Cascade Classifier Wandong Zhang1,2 · Qingzhong Li1 · Q. M. Jonathan Wu2 · Yimin Yang3 · Ming Li1 Received: 30 June 2018 / Accepted: 10 October 2018 / Published online: 24 October 2018 © Springer Science+Business Media, LLC, part of Springer Nature 2018 Abstract High-frequency surface wave radar (HFSWR) has a vital civilian and military significance for ship target detection and tracking because of its wide visual field and large sea area coverage. However, most of the existing ship target detection methods of HFSWR face two main difficulties: (1) the received radar signals are strongly polluted by clutter and noises, and (2) it is difficult to detect ship targets in real-time due to high computational complexity. This paper presents a ship target detection algorithm to overcome the problems above by using a two-stage cascade classification structure. Firstly, to quickly obtain the target candidate regions, a simple gray-scale feature and a linear classifier were applied. Then, a new error self-adjustment extreme learning machine (ES-ELM) with Haar-like input features was adopted to further identify the target precisely in each candidate region. The proposed ES-ELM includes two parts: initialization part and updating part. In the former stage, the L1 regularizer process is adopted to find the sparse solution of output weights, to prune the useless neural nodes and to obtain the optimal number of hidden neurons. Also, to ensure an excellent generalization performance by the network, in the latter stage, the parameters of hidden layer are updated through several iterations using L2 regularizer process with pulled back error matrix. -
Transmitter T (X) Channel E (X) Receiver R
Analog Communication ( 10EC53 ) Introduction The function of the communication system is to make available at the destination a signal originating at a distant point. This signal is called the desired signal. Unfortunately, during the passage of the signal through the channel and front-end of the receiver, this desired signal gets corrupted by a number of undesired signals. This is referred to as Noise. Noise is any unknown or unwanted signal. Noise is the static you hear in the speaker when you tune any AM or FM receiver to any position between stations. It is also the snow or confetti that is visible on a TV screen. The received signal is modeled as r (t) = s (t) + n (t) Where s (t) is the transmitted/desired signal n (t) is the additive noise/unwanted signal The signal n (t) gets added at the channel. It disturbs the transmission and processing of signal in Communication System. Over which one cannot have a control. In general term it is an unwanted signal that affects a wanted signal. It is a random signal that cannot be represented with a simple equation. But some time can be deterministic components (power supply hum, certain oscillations).Deterministic Components can be eliminated by proper shielding and introduction of notch filters. If their were no noise perfect communication would be possible with minimum transmitted power. At the receiver only amplification of the signal power to the desired level is required. If R (x)=T (x) then e (x)=0 Transmitter Receiver T (x) R (x) If R (x) ≠ T (x) then e (x) ≠ 0.The received signal is corrupted. -
Demodulation, Bit-Error Probability and Diversity Arrangements
RADIO SYSTEMS – ETIN15 Lecture no: 6 Demodulation, bit-error probability and diversity arrangements Anders J Johansson, Department of Electrical and Information Technology [email protected] 5 April 2017 1 Contents • Receiver noise calculations [Covered briefly in Chapter 3 of textbook!] • Optimal receiver and bit error probability – Principle of maximum-likelihood receiver – Error probabilities in non-fading channels – Error probabilities in fading channels • Diversity arrangements – The diversity principle – Types of diversity – Spatial (antenna) diversity performance 5 April 2017 2 RECEIVER NOISE 5 April 2017 3 Receiver noise Noise sources The noise situation in a receiver depends on several noise sources Noise picked up Wanted by the antenna signal Output signal Analog Detector with requirement circuits on quality Thermal noise 5 April 2017 4 Receiver noise Equivalent noise source To simplify the situation, we replace all noise sources with a single equivalent noise source. Wanted How do we determine Noise free signal N from the other sources? N Output signal C Analog Detector with requirement circuits on quality Noise free Same “input quality”, signal-to-noise ratio, C/N in the whole chain. 5 April 2017 5 Receiver noise Examples • Thermal noise is caused by random movements of electrons in circuits. It is assumed to be Gaussian and the power is proportional to the temperature of the material, in Kelvin. • Atmospheric noise is caused by electrical activity in the atmosphere, e.g. lightning. This noise is impulsive in its nature and below 20 MHz it is a dominating. • Cosmic noise is caused by radiation from space and the sun is a major contributor. -
Signal to Noise Ratio (SNR Or S/N) SNR Is the Ratio of the Signal Power to Noise Power
JAWAHARLAL NEHRU TECHNOLOGICAL UNIVERSITY KAKINADA KAKINADA – 533 001 , ANDHRA PRADESH GATE Coaching Classes as per the Direction of Ministry of Education GOVERNMENT OF ANDHRA PRADESH Analog Communication 26-05-2020 to 06-07-2020 Prof. Ch. Srinivasa Rao Dept. of ECE, JNTUK-UCE Vizianagaram Analog Communication-Day 7, 01-06-2020 Presentation Outline Noise: • Noise and its types • Thermal Noise • Parameters of Noise • Noise in Baseband Communication systems • Gaussian process and NB Noise • Problems 02-06-2020 Prof.Ch.Srinivasa Rao, JNTUK UCEV 2 Learning Outcomes • At the end of this Session, Student will be able to: • LO 1 : Fundamental aspects of Noise • LO 2 : Different Noise types and its Paraneters • LO 3 : Gaussian Process and ND Noise 02-06-2020 Prof.Ch.Srinivasa Rao, JNTUK UCEV 3 Noise Definitions of Noise: • Noise is unwanted signal that affects wanted signal. • Noise can broadly be defined as any unknown signal that affects the recovery of the desired signal. • Noise is an unwanted signal, which interferes with the original message signal and corrupts the parameters of the message signal. This alteration in the communication process, leads to the message getting altered. It most likely enters at the channel or the receiver. Effect of noise • Degrades system performance (Analog and digital) • Receiver cannot distinguish signal from noise • Efficiency of communication system reduces Most common examples of noise are: • Hiss sound in radio receivers • Buzz sound amidst of telephone conversations • Flicker in television receivers, Noise Sources External Internal Noise Noise Atmospheric Industrial Extraterrestrial Solar Noise Cosmic Noise External Noise : • It is due to Man- made and natural resources • Sources over which we have no control such as thunders, snow fall, lightning etc. -
Chapter 10 IONOSPHERICRADIO WAVE PROPAGATION Section 10.1 S
Chapter 10 IONOSPHERICRADIO WAVE PROPAGATION Section 10.1 S. Basu, J. Buchau, F.J. Rich and E.J. Weber Section 10.2 E.C. Field, J.L. Heckscher, P.A. Kossey, and E.A. Lewis Section 10.3 B.S. Dandekar Section 10.4 L.F. McNamara Section 10.5 E.W. Cliver Section 10.6 G.H. Millman Section 10.7 J. Aarons and S. Basu Section 10.8 J.A. Klobuchar Section 10.9 J.A. Klobuchar Section 10.10 S. Basu, M.F. Mendillo The series of reviews presented is an attempt to introduce in HF communications is leading to a rejuvenation of the ionospheric radio wave propagation of interest to system global ionosonde network. users. Although the attempt is made to summarize the field, the individuals writing each section have oriented the work 10.1.1.1 Ionogram. Ionospheric sounders or ionosondes in the direction judged to be most important. are, in principle, HF radars that record the time of flight or We cover areas such as HF and VLF propagation where travel of a transmitted HF signal as a measure of its ionos- the ionosphere is essentially a "black box", that is, a vital pheric reflection height. By sweeping in frequency, typically part of the system. We also cover areas where the ionosphere from 0.5 to 20 MHz, an ionosonde obtains a meas- is essentially a nuisance, such as the scintillations of trans- urement of the ionospheric reflection height as a function ionospheric radio signals. of frequency. A recording of this reflection height meas- Finally, we have included a summary of the main fea- urement as a function of frequency is called an ionogram. -
CALIFORNIA STATE UNIVERSITY, NORTHRIDGE SPACE COMMUNICATION THEORY and PRACTICE a Graduate Project Submitted in Partial Satisfac
CALIFORNIA STATE UNIVERSITY, NORTHRIDGE SPACE COMMUNICATION THEORY AND PRACTICE A graduate project submitted in partial satisfaction of the requirements for the degree of the Masterr of Science 1n Engineering by Kristine Patricia Maine January, 1983 The project of Kristine Patricia Maine is approved: CDr. Ste~en A. Gad~ski) (Dr. ~y H. Pettit, Chair) California State University, Northridge ii ACKNOWLEDGEMENTS A deep sense of gratitude is expressed to my academic advisor, Dr. Ray Pettit, for his invaluable counsel and advisement during the course of this study. In addition, I express thanks to The Aerospace Corporation for the use of their facilities and personnel for the completion of this document. iii TABLE OF CONTENTS Title Page Acknowledgement iii Table of Figures v List of Tables vii List of Symbols viii Abstract xi I. Introduction 1 II. Space Communication System Components 2 III. Theory of Link Analysis 8 IV. Applications of Link Analysis 21 v. Summary 41 Appendix A--Signal Losses 45 Appendix B--Space Shuttle Design Model 54 Appendix C--Communication Satellite Systems 62 Footnotes 67 Bi biliography 70 iv TABLE OF FIGURES Figure Number Title 1 Simplified Transponder Block Diagram 3 Showing a Single Channel Transponder 2 TWT Characteristics 4 3 Typical Antenna Beamwidth Pattern 7 4 Satellite Communication System 9 5 Uplink Model 10 6 Downlink Model 13 7 Satellite Communication Transmitter 16 to-Receiver with Typical Loss and Noise Sources 8 Zenith Attenuation Values Expected to 18 be Exceeded 2% of the Year at Mid latitudes 9 Probability -
Dr. Dharmendra Kumar Assistant Professor Department of Electronics and Communication Engineering MMM University of Technology, Gorakhpur–273010
Dr. Dharmendra Kumar Assistant Professor Department of Electronics and Communication Engineering MMM University of Technology, Gorakhpur–273010. Content of Unit-3 Noise: Source of Noise, Frequency domain, Representation of noise, Linear Filtering of noise, Noise in Amplitude modulation system, Noise in SSB-SC,DSB and DSB-C, Noise Ratio, Noise Comparison of FM and AM, Pre- emphasis and De-emphasis, Figure of Merit. Claude E. Shannon conceptualized the communication theory model in the late 1940s. It remains central to communication study today. Noise is random signal that exists in communication systems Channel is the main source of noise in communication systems Transmitter or Receiver may also induce noise in the system There are mainly 2-types of noise sources Internal noise source (are mainly internal to the communication system) External noise source Noise is an inconvenient feature which affects the system performance. Following are the effects of noise Degrade system performance for both analog and digital systems Noise limits the operating range of the systems Noise indirectly places a limit on the weakest signal that can be amplified by an amplifier. The oscillator in the mixer circuit may limit its frequency because of noise. A system’s operation depends on the operation of its circuits. Noise limits the smallest signal that a receiver is capable of processing The receiver can not understand the sender the receiver can not function as it should be. Noise affects the sensitivity of receivers: Sensitivity is the minimum amount of input signal necessary to obtain the specified quality output. Noise affects the sensitivity of a receiver system, which eventually affects the output. -
Impact of Power Line Telecommunication Systems on Radiocommunication Systems Operating in the LF, MF, HF and VHF Bands Below 80 Mhz
Report ITU-R SM.2158 (09/2009) Impact of power line telecommunication systems on radiocommunication systems operating in the LF, MF, HF and VHF bands below 80 MHz SM Series Spectrum management ii Rep. ITU-R SM.2158 Foreword The role of the Radiocommunication Sector is to ensure the rational, equitable, efficient and economical use of the radio-frequency spectrum by all radiocommunication services, including satellite services, and carry out studies without limit of frequency range on the basis of which Recommendations are adopted. The regulatory and policy functions of the Radiocommunication Sector are performed by World and Regional Radiocommunication Conferences and Radiocommunication Assemblies supported by Study Groups. Policy on Intellectual Property Right (IPR) ITU-R policy on IPR is described in the Common Patent Policy for ITU-T/ITU-R/ISO/IEC referenced in Annex 1 of Resolution ITU-R 1. Forms to be used for the submission of patent statements and licensing declarations by patent holders are available from http://www.itu.int/ITU-R/go/patents/en where the Guidelines for Implementation of the Common Patent Policy for ITU-T/ITU-R/ISO/IEC and the ITU-R patent information database can also be found. Series of ITU-R Reports (Also available online at http://www.itu.int/publ/R-REP/en) Series Title BO Satellite delivery BR Recording for production, archival and play-out; film for television BS Broadcasting service (sound) BT Broadcasting service (television) F Fixed service M Mobile, radiodetermination, amateur and related satellite services P Radiowave propagation RA Radio astronomy RS Remote sensing systems S Fixed-satellite service SA Space applications and meteorology SF Frequency sharing and coordination between fixed-satellite and fixed service systems SM Spectrum management Note: This ITU-R Report was approved in English by the Study Group under the procedure detailed in Resolution ITU-R 1. -
Annual Report 1955 National Bureau of Standards
Annual Report 1955 National Bureau of Standards U. S. Department of Commerce UNITED STATES DEPARTMENT OF COMMERCE Sinclair Weeks, Secretary NATIONAL BUREAU OF STANDARDS A. V. Astin, Director Annual Report 1955 National Bureau of Standards Miscellaneous Publication 217 For sale by the Superintendent of Documents, U. S. Government Printing Office Washington 25, D. C. - Price 55 cents Contents Page General Review 1 1.1. Introduction 1 1.2. Technical Activities 2 1.3. Administrative Activities 9 1.4. Publications 11 Research and Development Program 12 2.1. Electricity and Electronics 12 Fundamental electrical units 13, Electrochemistry 14, Improve- ments in measuring techniques 14, Resistor noise 15, Electron tubes 15, Research on electric spark discharge 16, Pole-top failure 16, Design of mutual inductance transducers 16, Marine weather station 16, Special electronic devices 17, Preferred circuits 17, Mechanized production of electronics 18. 2.2. Optics and Metrology 19 Intercomparison of standards 20, Determination of color dif- ferences 20, Glass color standards 21, Spectacle lenses 22, Aviation lighting 23, Refractometry of synthetic crystals 24. 2.3. Heat and Power 24 Temperature standards 25, Low-temperature research 26, Properties of air and related substances 28, Thermodynamic properties of metals and salts 29, Mechanical degradation of polymers 29, Combustion in engines 30. 2.4. Atomic and Radiation Physics 31 Velocity of light redetermined 31, Radiation balance 32, X-ray calorimeter 32, Electron beam extractor 33, New radiochem- istry laboratory 33, Attenuation of gamma rays at oblique incidence 34, Zone melting apparatus 35, International inter- comparison of radiation standards 36, Negative ion research 36. 2.5. -
General Disclaimer One Or More of the Following Statements May Affect
General Disclaimer One or more of the Following Statements may affect this Document This document has been reproduced from the best copy furnished by the organizational source. It is being released in the interest of making available as much information as possible. This document may contain data, which exceeds the sheet parameters. It was furnished in this condition by the organizational source and is the best copy available. This document may contain tone-on-tone or color graphs, charts and/or pictures, which have been reproduced in black and white. This document is paginated as submitted by the original source. Portions of this document are not fully legible due to the historical nature of some of the material. However, it is the best reproduction available from the original submission. Produced by the NASA Center for Aerospace Information (CASI) UNIVERSITY OF ILLINOIS URBANA AERONOMY REPfC".)IRT NO, 67 1 AN INVESTIGATION OF THE SOLAR ZENITH ANGLE VARIATION OF D-REGION IONIZATION (NASA-CF-143217) AN INVE'STIGATICN CF THE N75 -28597 SOIA; ZENITH ANGLE VAFIATICN CF C-FEGICN IONI2ATICN (I11incis Cniv.) 290 F HC iE.75 CSCL 044 U11C13s G3/4b 31113 by ^' x '^^ P. A. J. Ratnasiri o c n C. F. Scchrist, Jr. m y T April I, 1975 Library of Congress ISSN 0568-0581 Aeronomy Laboratory Supported by Department of Electrical Engineering National Science Foundation University of Illinois Grant GA 3691 IX Urbana, Illinois ii ABSTRACT A review of the D- region ionization measurements and its solar zenith angle variation reveals that a unified model of the D region, incorporating both thenetural chemistry and the ion chemistry, is required for a proper understanding of this region of the ionosphere. -
Design Patterns and Software Techniques for Large-Scale, Open and Reproducible Data Reduction
DESIGN PATTERNS AND SOFTWARE TECHNIQUES FOR LARGE-SCALE, OPEN AND REPRODUCIBLE DATA REDUCTION by Gijs Jan Molenaar A thesis submitted in fulfilment of the requirements for the degree of Doctor of Philosophy Supervised by Prof. Oleg Smirnov 2021 Contents Abstract v Declaration of Authorship vii Acknowledgements viii Preface x Publications xi Source Code xii 1 History of data reduction pipelines 1 1.1 Introduction . .2 1.2 1930s . .2 1.3 1940s . .2 1.4 1950s . .5 1.5 1960s . .5 1.6 1970s . .7 Zero-generation calibration to first-generation calibration . .8 Astronomical Image Processing System . 10 CANDID . 10 CLEAN . 11 1.7 1980s . 11 Self-calibration and the second generation of calibration algorithms . 13 GIPSY . 13 DWARF..................................... 14 IRAF ...................................... 15 Miriad . 15 1.8 Meanwhile in South Africa . 15 1.9 1990s . 17 AIPS++ . 17 NEWSTAR . 18 The Measurement Set version 1 . 18 The Measurement equation . 19 DIFMAP . 19 1.10 2000s . 19 The Measurement Set version 2 . 19 i CONTENTS ii MeqTrees, third-generation calibration algorithms . 19 Obit....................................... 19 CASA...................................... 20 Casacore . 20 1.11 2010s . 21 Cuisine . 21 Docker...................................... 21 The ALMA pipeline . 21 Common Workflow Language . 22 DDFacet and killMS . 22 Kliko....................................... 22 Stimela . 23 CARACal (formerly known as MeerKATHI) . 23 Default pre-processing pipeline . 23 The LOFAR two-metre survey pipeline . 24 1.12 Overview of events . 25 1.13 Discussion . 27 2 Fundamentals 29 2.1 Electromagnetic radiation . 30 2.2 Interferometry . 33 Aperture synthesis . 34 The (u; v; w) coordinate system . 36 The radio interferometer measurement equation . 37 2.3 Image reconstruction .