Phase Reddening on Near-Earth Asteroids: Implications For
Total Page:16
File Type:pdf, Size:1020Kb
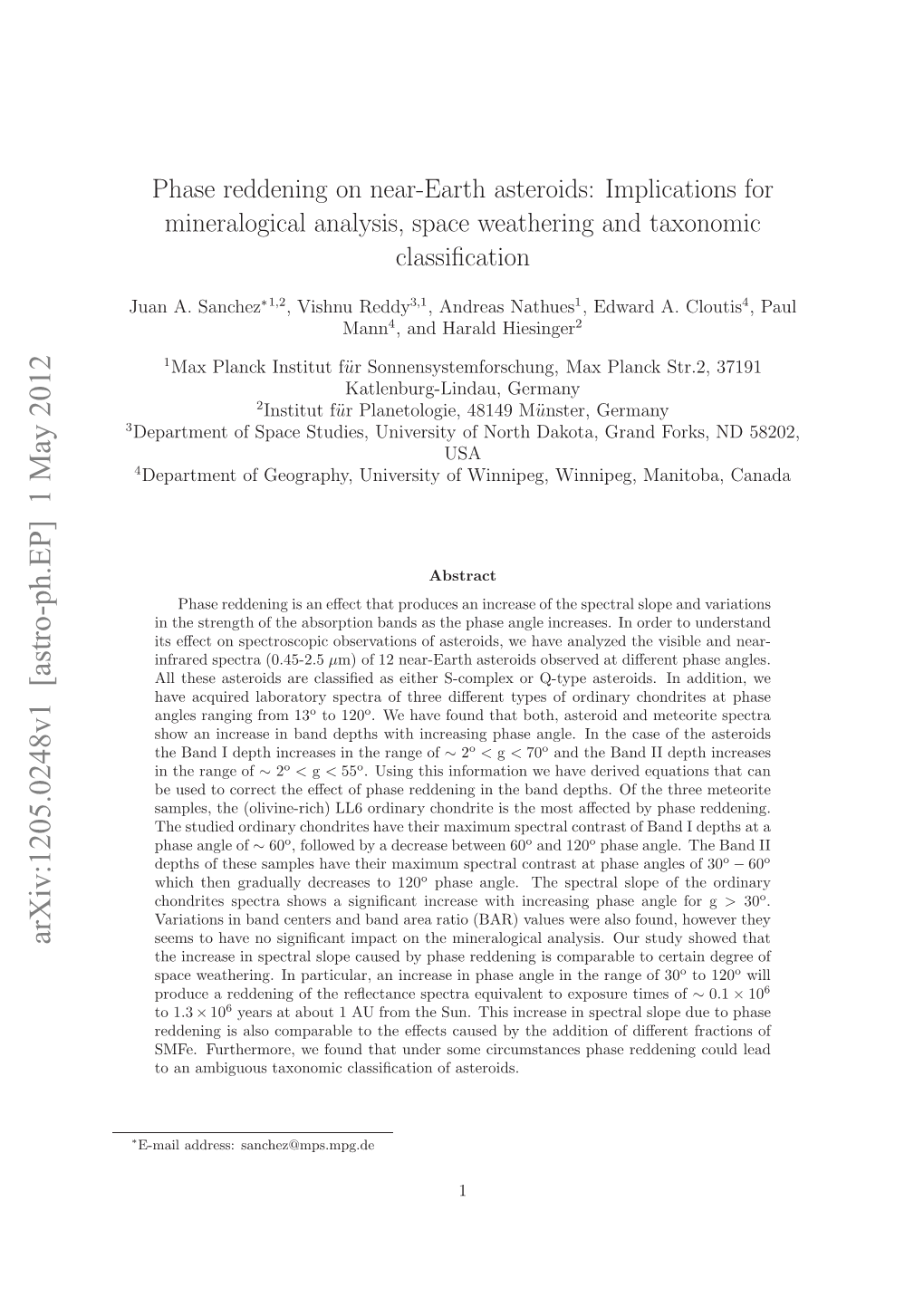
Load more
Recommended publications
-
The Minor Planet Bulletin
THE MINOR PLANET BULLETIN OF THE MINOR PLANETS SECTION OF THE BULLETIN ASSOCIATION OF LUNAR AND PLANETARY OBSERVERS VOLUME 36, NUMBER 3, A.D. 2009 JULY-SEPTEMBER 77. PHOTOMETRIC MEASUREMENTS OF 343 OSTARA Our data can be obtained from http://www.uwec.edu/physics/ AND OTHER ASTEROIDS AT HOBBS OBSERVATORY asteroid/. Lyle Ford, George Stecher, Kayla Lorenzen, and Cole Cook Acknowledgements Department of Physics and Astronomy University of Wisconsin-Eau Claire We thank the Theodore Dunham Fund for Astrophysics, the Eau Claire, WI 54702-4004 National Science Foundation (award number 0519006), the [email protected] University of Wisconsin-Eau Claire Office of Research and Sponsored Programs, and the University of Wisconsin-Eau Claire (Received: 2009 Feb 11) Blugold Fellow and McNair programs for financial support. References We observed 343 Ostara on 2008 October 4 and obtained R and V standard magnitudes. The period was Binzel, R.P. (1987). “A Photoelectric Survey of 130 Asteroids”, found to be significantly greater than the previously Icarus 72, 135-208. reported value of 6.42 hours. Measurements of 2660 Wasserman and (17010) 1999 CQ72 made on 2008 Stecher, G.J., Ford, L.A., and Elbert, J.D. (1999). “Equipping a March 25 are also reported. 0.6 Meter Alt-Azimuth Telescope for Photometry”, IAPPP Comm, 76, 68-74. We made R band and V band photometric measurements of 343 Warner, B.D. (2006). A Practical Guide to Lightcurve Photometry Ostara on 2008 October 4 using the 0.6 m “Air Force” Telescope and Analysis. Springer, New York, NY. located at Hobbs Observatory (MPC code 750) near Fall Creek, Wisconsin. -
Temperature-Induced Effects and Phase Reddening on Near-Earth Asteroids
Planetologie Temperature-induced effects and phase reddening on near-Earth asteroids Inaugural-Dissertation zur Erlangung des Doktorgrades der Naturwissenschaften im Fachbereich Geowissenschaften der Mathematisch-Naturwissenschaftlichen Fakultät der Westfälischen Wilhelms-Universität Münster vorgelegt von Juan A. Sánchez aus Caracas, Venezuela -2013- Dekan: Prof. Dr. Hans Kerp Erster Gutachter: Prof. Dr. Harald Hiesinger Zweiter Gutachter: Dr. Vishnu Reddy Tag der mündlichen Prüfung: 4. Juli 2013 Tag der Promotion: 4. Juli 2013 Contents Summary 5 Preface 7 1 Introduction 11 1.1 Asteroids: origin and evolution . 11 1.2 The asteroid-meteorite connection . 13 1.3 Spectroscopy as a remote sensing technique . 16 1.4 Laboratory spectral calibration . 24 1.5 Taxonomic classification of asteroids . 31 1.6 The NEA population . 36 1.7 Asteroid space weathering . 37 1.8 Motivation and goals of the thesis . 41 2 VNIR spectra of NEAs 43 2.1 The data set . 43 2.2 Data reduction . 45 3 Temperature-induced effects on NEAs 55 3.1 Introduction . 55 3.2 Temperature-induced spectral effects on NEAs . 59 3.2.1 Spectral band analysis of NEAs . 59 3.2.2 NEAs surface temperature . 59 3.2.3 Temperature correction to band parameters . 62 3.3 Results and discussion . 70 4 Phase reddening on NEAs 73 4.1 Introduction . 73 4.2 Phase reddening from ground-based observations of NEAs . 76 4.2.1 Phase reddening effect on the band parameters . 76 4.3 Phase reddening from laboratory measurements of ordinary chondrites . 82 4.3.1 Data and spectral band analysis . 82 4.3.2 Phase reddening effect on the band parameters . -
1620 Geographos and 433 Eros: Shaped by Planetary Tides?
View metadata, citation and similar papers at core.ac.uk brought to you by CORE provided by CERN Document Server 1620 Geographos and 433 Eros: Shaped by Planetary Tides? W. F. Bottke, Jr. Center for Radiophysics and Space Research, Cornell University, Ithaca, NY 14853-6801 D. C. Richardson Department of Astronomy, University of Washington, Box 351580, Seattle, WA 98195 P. Michel Osservatorio Astronomico di Torino, Strada Osservatorio 20, 10025 Pino Torinese (TO), Italy and S. G. Love Jet Propulsion Laboratory, California Institute of Technology, M/S 306-438, 4800 Oak Grove Drive, Pasadena, CA 91109-8099 Received 23 September 1998; accepted 10 December 1998 –2– ABSTRACT Until recently, most asteroids were thought to be solid bodies whose shapes were determined largely by collisions with other asteroids (Davis et al., 1989). It now seems that many asteroids are little more than rubble piles, held together by self-gravity (Burns 1998); this means that their shapes may be strongly distorted by tides during close encounters with planets. Here we report on numerical simulations of encounters between a ellipsoid-shaped rubble-pile asteroid and the Earth. After an encounter, many of the simulated asteroids develop the same rotation rate and distinctive shape (i.e., highly elongated with a single convex side, tapered ends, and small protuberances swept back against the rotation direction) as 1620 Geographos. Since our numerical studies show that these events occur with some frequency, we suggest that Geographos may be a tidally distorted object. In addition, our work shows that 433 Eros, which will be visited by the NEAR spacecraft in 1999, is much like Geographos, which suggests that it too may have been molded by tides in the past. -
Physical Properties of Near-Earth Asteroids
Planet. Space Sci., Vol. 46, No. 1, pp. 47-74, 1998 Pergamon N~I1998 Elsevier Science Ltd All rights reserved. Printed in Great Britain 00324633/98 $19.00+0.00 PII: SOO32-0633(97)00132-3 Physical properties of near-Earth asteroids D. F. Lupishko’ and M. Di Martino’ ’ Astronomical Observatory of Kharkov State University, Sumskaya str. 35, Kharkov 310022, Ukraine ‘Osservatorio Astronomic0 di Torino, I-10025 Pino Torinese (TO), Italy Received 5 February 1997; accepted 20 June 1997 rather small objects, usually of the order of a few kilo- metres or less. MBAs of such sizes are generally not access- ible to ground-based observations. Therefore, when NEAs approach the Earth (at distances which can be as small as 0.01-0.02 AU and sometimes less) they give a unique chance to study objects of such small sizes. Some of them possibly represent primordial matter, which has preserved a record of the earliest stages of the Solar System evolution, while the majority are fragments coming from catastrophic collisions that occurred in the asteroid main- belt and could provide “a look” at the interior of their much larger parent bodies. Therefore, NEAs are objects of special interest for sev- eral reasons. First, from the point of view of fundamental science, the problems raised by their origin in planet- crossing orbits, their life-time, their possible genetic relations with comets and meteorites, etc. are closely connected with the solution of the major problem of “We are now on the threshold of a new era of asteroid planetary science of the origin and evolution of the Solar studies” System. -
Observations from Orbiting Platforms 219
Dotto et al.: Observations from Orbiting Platforms 219 Observations from Orbiting Platforms E. Dotto Istituto Nazionale di Astrofisica Osservatorio Astronomico di Torino M. A. Barucci Observatoire de Paris T. G. Müller Max-Planck-Institut für Extraterrestrische Physik and ISO Data Centre A. D. Storrs Towson University P. Tanga Istituto Nazionale di Astrofisica Osservatorio Astronomico di Torino and Observatoire de Nice Orbiting platforms provide the opportunity to observe asteroids without limitation by Earth’s atmosphere. Several Earth-orbiting observatories have been successfully operated in the last decade, obtaining unique results on asteroid physical properties. These include the high-resolu- tion mapping of the surface of 4 Vesta and the first spectra of asteroids in the far-infrared wave- length range. In the near future other space platforms and orbiting observatories are planned. Some of them are particularly promising for asteroid science and should considerably improve our knowledge of the dynamical and physical properties of asteroids. 1. INTRODUCTION 1800 asteroids. The results have been widely presented and discussed in the IRAS Minor Planet Survey (Tedesco et al., In the last few decades the use of space platforms has 1992) and the Supplemental IRAS Minor Planet Survey opened up new frontiers in the study of physical properties (Tedesco et al., 2002). This survey has been very important of asteroids by overcoming the limits imposed by Earth’s in the new assessment of the asteroid population: The aster- atmosphere and taking advantage of the use of new tech- oid taxonomy by Barucci et al. (1987), its recent extension nologies. (Fulchignoni et al., 2000), and an extended study of the size Earth-orbiting satellites have the advantage of observing distribution of main-belt asteroids (Cellino et al., 1991) are out of the terrestrial atmosphere; this allows them to be in just a few examples of the impact factor of this survey. -
Asteroid Regolith Weathering: a Large-Scale Observational Investigation
University of Tennessee, Knoxville TRACE: Tennessee Research and Creative Exchange Doctoral Dissertations Graduate School 5-2019 Asteroid Regolith Weathering: A Large-Scale Observational Investigation Eric Michael MacLennan University of Tennessee, [email protected] Follow this and additional works at: https://trace.tennessee.edu/utk_graddiss Recommended Citation MacLennan, Eric Michael, "Asteroid Regolith Weathering: A Large-Scale Observational Investigation. " PhD diss., University of Tennessee, 2019. https://trace.tennessee.edu/utk_graddiss/5467 This Dissertation is brought to you for free and open access by the Graduate School at TRACE: Tennessee Research and Creative Exchange. It has been accepted for inclusion in Doctoral Dissertations by an authorized administrator of TRACE: Tennessee Research and Creative Exchange. For more information, please contact [email protected]. To the Graduate Council: I am submitting herewith a dissertation written by Eric Michael MacLennan entitled "Asteroid Regolith Weathering: A Large-Scale Observational Investigation." I have examined the final electronic copy of this dissertation for form and content and recommend that it be accepted in partial fulfillment of the equirr ements for the degree of Doctor of Philosophy, with a major in Geology. Joshua P. Emery, Major Professor We have read this dissertation and recommend its acceptance: Jeffrey E. Moersch, Harry Y. McSween Jr., Liem T. Tran Accepted for the Council: Dixie L. Thompson Vice Provost and Dean of the Graduate School (Original signatures are on file with official studentecor r ds.) Asteroid Regolith Weathering: A Large-Scale Observational Investigation A Dissertation Presented for the Doctor of Philosophy Degree The University of Tennessee, Knoxville Eric Michael MacLennan May 2019 © by Eric Michael MacLennan, 2019 All Rights Reserved. -
ASTEROID SPECTROSCOPY and MINERALOGY 8:30 A.M
Lunar and Planetary Science XXXVI (2005) sess53.pdf Wednesday, March 16, 2005 ASTEROID SPECTROSCOPY AND MINERALOGY 8:30 a.m. Salon A Chairs: S. Erard L. A. McFadden 8:30 a.m. Gaffey M. J. * The Critical Importance of Data Reduction Calibrations in the Interpretability of S-type Asteroid Spectra [#1916] S-asteroid spectra are especially sensitive to distortion during the reduction of raw data to calibrated spectra. This can lead to major errors in mineralogical interpretations. Two major problems and the procedures to ameliorate them are discussed. 8:45 a.m. Sunshine J. M. * Bus S. J. Burbine T. H. McCoy T. J. Tracing Oxygen Fugacity in Asteroids and Meteorites Through Olivine Composition [#1203] We present new spectra of well-characterized olivine-rich meteorites and show that olivine Fa# can be accurately inferred from spectra. Using the same approach, new asteroid data are examined to infer the oxygen fugacity of olivine-rich asteroids. 9:00 a.m. Trigo-Rodríguez J. M. * Castro-Tirado A. J. Llorca J. Evidence of Hydrated 109P/Swift-Tuttle Meteoroids from Meteor Spectroscopy [#1485] Evidence for the possible presence of water in meteoroids released from comet 109P/Swift-Tuttle is presented. A Perseid fireball spectrum obtained during the 2004 campaign shows O and H lines, consistent with the presence of water in the mineral components of the meteoroid. 9:15 a.m. Emery J. P. * Cruikshank D. P. Van Cleve J. Stansberry J. A. Mineralogy of Asteroids from Observations with the Spitzer Space Telescope [#2072] Thermal emission measurements of the low-albedo Trojan asteroid 624 Hektor indicate a surface mineralogy of fine-grained silicates. -
~XECKDING PAGE BLANK WT FIL,,Q
1,. ,-- ,-- ~XECKDING PAGE BLANK WT FIL,,q DYNAMICAL EVIDENCE REGARDING THE RELATIONSHIP BETWEEN ASTEROIDS AND METEORITES GEORGE W. WETHERILL Department of Temcltricrl kgnetism ~amregie~mtittition of Washington Washington, D. C. 20025 Meteorites are fragments of small solar system bodies (comets, asteroids and Apollo objects). Therefore they may be expected to provide valuable information regarding these bodies. How- ever, the identification of particular classes of meteorites with particular small bodies or classes of small bodies is at present uncertain. It is very unlikely that any significant quantity of meteoritic material is obtained from typical ac- tive comets. Relatively we1 1-studied dynamical mechanisms exist for transferring material into the vicinity of the Earth from the inner edge of the asteroid belt on an 210~-~year time scale. It seems likely that most iron meteorites are obtained in this way, and a significant yield of complementary differec- tiated meteoritic silicate material may be expected to accom- pany these differentiated iron meteorites. Insofar as data exist, photometric measurements support an association between Apollo objects and chondri tic meteorites. Because Apol lo ob- jects are in orbits which come close to the Earth, and also must be fragmented as they traverse the asteroid belt near aphel ion, there also must be a component of the meteorite flux derived from Apollo objects. Dynamical arguments favor the hypothesis that most Apollo objects are devolatilized comet resiaues. However, plausible dynamical , petrographic, and cosmogonical reasons are known which argue against the simple conclusion of this syllogism, uiz., that chondri tes are of cometary origin. Suggestions are given for future theoretical , observational, experimental investigations directed toward improving our understanding of this puzzling situation. -
1987Aj 94. . 18 9Y the Astronomical Journal
9Y 18 . THE ASTRONOMICAL JOURNAL VOLUME 94, NUMBER 1 JULY 1987 94. RADAR ASTROMETRY OF NEAR-EARTH ASTEROIDS D. K. Yeomans, S. J. Ostro, and P. W. Chodas Jet Propulsion Laboratory, California Institute of Technology, 4800 Oak Grove Drive, Pasadena, California 91109 1987AJ Received 26 January 1987; revised 14 March 1987 ABSTRACT In an effort to assess the extent to which radar observations can improve the accuracy of near-Earth asteroid ephemerides, an uncertainty analysis has been conducted using four asteroids with different histories of optical and radar observations. We selected 1627 Ivar as a representative numbered asteroid with a long (56 yr) optical astrometric history and a very well-established orbit. Our other case studies involve three unnumbered asteroids (1986 DA, 1986 JK, and 1982 DB), whose optical astrometric histories are on the order of months and whose orbits are relatively poorly known. In each case study, we assumed that radar echoes obtained during the asteroid’s most recent close approach to Earth yielded one or more time-delay (distance) and/or Doppler-frequency (radial-velocity) estimates. We then studied the sensitivity of the asteroid’s predicted ephemeris uncertainties to the history (the sched- ule and accuracy) of the radar measurements as well as to the optical astrometric history. For any given data set of optical and radar observations and their associated errors, we calculated the angular (plane- of-sky) and Earth-asteroid distance uncertainties as functions of time through 2001. The radar data provided only a modest absolute improvement for the case when a long history of optical astrometric data exists (1627 Ivar), but rather dramatic reductions in the future ephemeris uncertainties of aster- oids having only short optical-data histories. -
Radar Observations of Asteroid 1620 Geographos
ICARUS 121, 46±66 (1996) ARTICLE NO. 0071 Radar Observations of Asteroid 1620 Geographos STEVEN J. OSTRO,RAYMOND F. JURGENS, AND KEITH D. ROSEMA Jet Propulsion Laboratory, California Institute of Technology, Pasadena, California 91109±8099 E-mail: [email protected] R. SCOTT HUDSON School of Electrical Engineering and Computer Science, Washington State University, Pullman, Washington 99164±2752 AND JON D. GIORGINI,RON WINKLER,DONALD K. YEOMANS,DENNIS CHOATE,RANDY ROSE,MARTIN A. SLADE, S. DENISE HOWARD,DANIEL J. SCHEERES, AND DAVID L. MITCHELL Jet Propulsion Laboratory, Pasadena, California 91109±8099 Received July 31, 1995; revised October 30, 1995 1. INTRODUCTION Goldstone radar observations of Geographos from August 28 through September 2, 1994 yield over 400 delay-Doppler images whose linear spatial resolutions range from p75 to Asteroid 1620 Geographos was discovered in 1951 by p151 m, and 138 pairs of dual-polarization (OC, SC) spectra A. G. Wilson and R. Minkowski and was observed over with one-dimensional resolution of 103 m. Each data type an eight-month interval in 1969 by Dunlap (1974) for ``light provides thorough rotational coverage. The images contain variation, colors, and polarization.'' From his analysis, an intrinsic north/south ambiguity, but the equatorial view which included experiments with laboratory models (Dun- allows accurate determination of the shape of the radar- lap 1972), he estimated the asteroid's spin vector and geo- facing part of the asteroid's pole-on silhouette at any rotation metric albedo, and noted that ``the best ®tting model (a phase. Sums of co-registered images that cover nearly a full cylinder with hemispherical ends) had a length to width rotation have de®ned the extremely elongated shape of that ratio of 2.7, which results in Geographos being 1.50 6 0.15 silhouette (S. -
Mineralogies and Source Regions of Near-Earth Asteroids ⇑ Tasha L
Icarus 222 (2013) 273–282 Contents lists available at SciVerse ScienceDirect Icarus journal homepage: www.elsevier.com/locate/icarus Mineralogies and source regions of near-Earth asteroids ⇑ Tasha L. Dunn a, , Thomas H. Burbine b, William F. Bottke Jr. c, John P. Clark a a Department of Geography-Geology, Illinois State University, Normal, IL 61790, United States b Department of Astronomy, Mount Holyoke College, South Hadley, MA 01075, United States c Southwest Research Institute, Boulder, CO 80302, United States article info abstract Article history: Near-Earth Asteroids (NEAs) offer insight into a size range of objects that are not easily observed in the Received 8 October 2012 main asteroid belt. Previous studies on the diversity of the NEA population have relied primarily on mod- Revised 8 November 2012 eling and statistical analysis to determine asteroid compositions. Olivine and pyroxene, the dominant Accepted 8 November 2012 minerals in most asteroids, have characteristic absorption features in the visible and near-infrared Available online 21 November 2012 (VISNIR) wavelengths that can be used to determine their compositions and abundances. However, formulas previously used for deriving compositions do not work very well for ordinary chondrite Keywords: assemblages. Because two-thirds of NEAs have ordinary chondrite-like spectral parameters, it is essential Asteroids, Composition to determine accurate mineralogies. Here we determine the band area ratios and Band I centers of 72 Meteorites Spectroscopy NEAs with visible and near-infrared spectra and use new calibrations to derive the mineralogies 47 of these NEAs with ordinary chondrite-like spectral parameters. Our results indicate that the majority of NEAs have LL-chondrite mineralogies. -
Orders of Magnitude (Length) - Wikipedia
03/08/2018 Orders of magnitude (length) - Wikipedia Orders of magnitude (length) The following are examples of orders of magnitude for different lengths. Contents Overview Detailed list Subatomic Atomic to cellular Cellular to human scale Human to astronomical scale Astronomical less than 10 yoctometres 10 yoctometres 100 yoctometres 1 zeptometre 10 zeptometres 100 zeptometres 1 attometre 10 attometres 100 attometres 1 femtometre 10 femtometres 100 femtometres 1 picometre 10 picometres 100 picometres 1 nanometre 10 nanometres 100 nanometres 1 micrometre 10 micrometres 100 micrometres 1 millimetre 1 centimetre 1 decimetre Conversions Wavelengths Human-defined scales and structures Nature Astronomical 1 metre Conversions https://en.wikipedia.org/wiki/Orders_of_magnitude_(length) 1/44 03/08/2018 Orders of magnitude (length) - Wikipedia Human-defined scales and structures Sports Nature Astronomical 1 decametre Conversions Human-defined scales and structures Sports Nature Astronomical 1 hectometre Conversions Human-defined scales and structures Sports Nature Astronomical 1 kilometre Conversions Human-defined scales and structures Geographical Astronomical 10 kilometres Conversions Sports Human-defined scales and structures Geographical Astronomical 100 kilometres Conversions Human-defined scales and structures Geographical Astronomical 1 megametre Conversions Human-defined scales and structures Sports Geographical Astronomical 10 megametres Conversions Human-defined scales and structures Geographical Astronomical 100 megametres 1 gigametre