Controlled-Source Seismic Reflection Interferometry
Total Page:16
File Type:pdf, Size:1020Kb
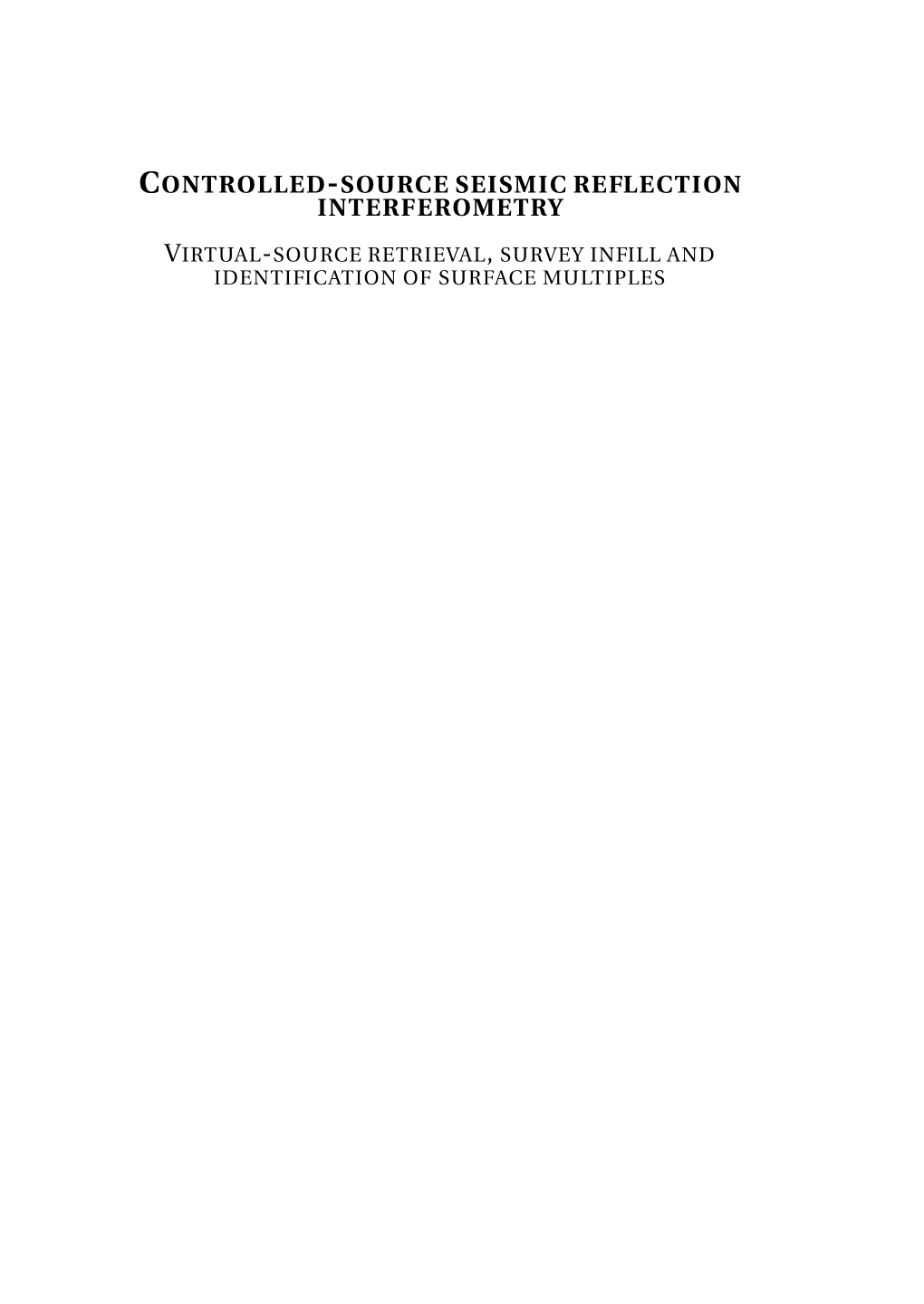
Load more
Recommended publications
-
Passive Seismic Interferometry in the Real World: Application with Microseismic and Traffic Noise
Passive seismic interferometry in the real world: Application with microseismic and traffic noise By Yang Zhao A dissertation submitted in partial satisfaction of the requirements for the degree of Doctor of Philosophy in Engineering - Civil and Environmental Engineering in the Graduate Division of the University of California, Berkeley Committee in charge: Professor James W. Rector III, Chair Professor Steven D. Glaser Professor Doug Dreger Fall 2013 Passive seismic interferometry in the real world: Application with microseismic and traffic noise © 2013 by Yang Zhao Abstract Passive seismic interferometry in the real world: Application with microseismic and traffic noise by Yang Zhao Doctor of Philosophy in Civil and Environmental Engineering University of California, Berkeley Professor James Rector III, Chair The past decade witnessed rapid development of the theory of passive seismic interferometry followed by numerous applications of interferometric approaches in seismic exploration and exploitation. Developments conclusively demonstrates that a stack of cross- correlations of traces recorded by two receivers over sources appropriately distributed in three-dimensional heterogeneous earth can retrieve a signal that would be observed at one receiver if another acted as a source of seismic waves. The main objective of this dissertation was to review the mathematical proof of passive seismic interferometry, and to develop innovative applications using microseismicity induced by hydraulic fracturing and near-surface void characterization. We began this dissertation with the definitions and mathematical proof of Green’s function representation, together with the description of the physical mechanisms of passive seismic interferometry. Selected computational methods of passive seismic interferometry are also included. The first application was to extract body waves and perform anisotropy analysis from passive downhole microseismic noise acquired in hydrocarbon-bearing reservoirs. -
Ambient Seismic Noise Imaging of the Lowermost Mantle Beneath the North Atlantic Ocean Lise Retailleau, Pierre Boué, Lei Li, Michel Campillo
Ambient Seismic Noise Imaging of the Lowermost Mantle Beneath the North Atlantic Ocean Lise Retailleau, Pierre Boué, Lei Li, Michel Campillo To cite this version: Lise Retailleau, Pierre Boué, Lei Li, Michel Campillo. Ambient Seismic Noise Imaging of the Lower- most Mantle Beneath the North Atlantic Ocean. Geophysical Journal International, Oxford University Press (OUP), 2020, 222 (2), pp.1339-1351. 10.1093/gji/ggaa210. hal-02928287 HAL Id: hal-02928287 https://hal.univ-grenoble-alpes.fr/hal-02928287 Submitted on 2 Sep 2020 HAL is a multi-disciplinary open access L’archive ouverte pluridisciplinaire HAL, est archive for the deposit and dissemination of sci- destinée au dépôt et à la diffusion de documents entific research documents, whether they are pub- scientifiques de niveau recherche, publiés ou non, lished or not. The documents may come from émanant des établissements d’enseignement et de teaching and research institutions in France or recherche français ou étrangers, des laboratoires abroad, or from public or private research centers. publics ou privés. Ambient Seismic Noise Imaging of the Lowermost Mantle Beneath the North Atlantic Ocean Lise Retailleau1,2*, Pierre Boué3, Lei Li3 and Michel Campillo3 1 Department of Geophysics, Stanford University, Stanford, California, USA. 2 Now at Observatoire Volcanologique du Piton de la Fournaise, Institut de Physique du Globe de Paris, CNRS, UMR 7154-Sismologie, La Plaine des Cafres, La Réunion, France 3 Université Grenoble Alpes, Univ. Savoie Mont Blanc, CNRS, IRD, IFSTTAR, ISTerre, 38000 -
Tutorial on Seismic Interferometry: Part 1 — Basic Principles and Applications
GEOPHYSICS, VOL.75, NO. 5 ͑SEPTEMBER-OCTOBER 2010͒; P.75A195–75A209, 15 FIGS. 10.1190/1.3457445 Tutorial on seismic interferometry: Part 1 — Basic principles and applications Kees Wapenaar1, Deyan Draganov1, Roel Snieder2, Xander Campman3, and Arie Verdel3 ABSTRACT retrieval of seismic surface-wave responses from ambient noise and the subsequent tomographic determination of the surface- Seismic interferometry involves the crosscorrelation of re- wave velocity distribution of the subsurface. Seismic interferom- sponses at different receivers to obtain the Green’s function be- etry is not restricted to retrieving direct waves between receivers. tween these receivers. For the simple situation of an impulsive In a classic paper, Claerbout shows that the autocorrelation of the plane wave propagating along the x-axis, the crosscorrelation of transmission response of a layered medium gives the plane-wave the responses at two receivers along the x-axis gives the Green’s reflection response of that medium. This is essentially 1D reflect- function of the direct wave between these receivers. When the ed-wave interferometry. Similarly, the crosscorrelation of the source function of the plane wave is a transient ͑as in exploration transmission responses, observed at two receivers, of an arbitrary seismology͒ or a noise signal ͑as in passive seismology͒, then the inhomogeneous medium gives the 3D reflection response of that crosscorrelation gives the Green’s function, convolved with the medium. One of the main applications of reflected-wave interfer- autocorrelation of the source function. Direct-wave interferome- ometry is retrieving the seismic reflection response from ambient try also holds for 2D and 3D situations, assuming the receivers noise and imaging of the reflectors in the subsurface. -
Seismic Interferometry: Reconstructing the Earth's Reflection Response
GEOPHYSICS, VOL.71, NO. 4 ͑JULY-AUGUST 2006͒; P.SI61–SI70, 17 FIGS. 10.1190/1.2209947 Seismic interferometry: Reconstructing the earth’s reflection response Deyan Draganov1, Kees Wapenaar1, and Jan Thorbecke1 ABSTRACT directions — the reconstructed reflection responses and the mi- grated depth image exhibit all the reflection events and the sub- In 1968, Jon Claerbout showed that the reflection response of a surface structures of interest, respectively. With only a few sub- 1D acoustic medium can be reconstructed by autocorrelating the surface sources, that is, with insufficient illumination, the recon- transmission response. Since then, several authors have derived structed reflection responses are noisy and can even become ki- relationships for reconstructing Green’s functions at the surface, nematically incorrect. At the same time, however, the depth using crosscorrelations of ͑noise͒ recordings that were taken at image, which was obtained from their migration, still shows the surface and that derived from subsurface sources. clearly all the illuminated subsurface structures at their correct For acoustic media, we review relations between the reflection positions. response and the transmission response in 3D inhomogeneous For the elastic case, we review a relationship between the re- lossless media. These relations are derived from a one-way flection Green’s functions and the transmission Green’s func- wavefield reciprocity theorem. We use modeling results to show tions derived from a two-way wavefield reciprocity theorem. Us- how to reconstruct the reflection response in the presence of tran- ing modeling examples, we show how to reconstruct the different sient subsurface sources with distinct excitation times, as well as components of the particle velocity observed at the surface and in the presence of simultaneously acting noise sources in the sub- resulting from a surface traction source. -
Analysis and Suppression of Passive Noise in Surface Microseismic Data
ANALYSIS AND SUPPRESSION OF PASSIVE NOISE IN SURFACE MICROSEISMIC DATA by Farnoush Forghani-Arani A thesis submitted to the Faculty and the Board of Trustees of the Colorado School of Mines in partial fulfillment of the requirements for the degree of Doctor of Philosophy (Geophysics). Golden, Colorado Date Signed: Farnoush Forghani-Arani Approved: Dr. Michael Batzle Professor of Geophysics Thesis Advisor Golden, Colorado Date Dr. Terence K. Young Professor and Head, Department of Geophysics ii ABSTRACT Surface microseismic surveys are gaining popularity in monitoring hydraulic fracturing processes. The effectiveness of these surveys, however, is strongly dependent on the signal- to-noise ratio of the acquired data. Cultural and industrial noise generated during hydraulic fracturing operations usually dominate the data, thereby decreasing the effectiveness of using these data in identifying and locating microseismic events. Hence, noise suppression is a crit- ical step in surface microseismic monitoring. In this thesis, I focus on two important aspects in using surface-recorded microseismic seismic data: first, I take advantage of the unwanted surface noise to understand the characteristics of these noise and extract information about the propagation medium from the noise; second, I propose effective techniques to suppress the surface noise while preserving the waveforms that contain information about the source of microseisms. Automated event identification on passive seismic data using only a few receivers is challenging especially when the record lengths span long durations of time. I introduce an automatic event identification algorithm that is designed specifically for detecting events in passive data acquired with a small number of receivers. I demonstrate that the conventional ST A/LT A (Short-term Average/Long-term Average) algorithm is not sufficiently effective in event detection in the common case of low signal-to-noise ratio. -
INVESTIGATION of AMBIENT SEISMIC NOISE USING SEISMIC INTERFEROMETRY in WESTERN MONTANA Natalia Krzywosz Montana Tech of the University of Montana
Montana Tech Library Digital Commons @ Montana Tech Graduate Theses & Non-Theses Student Scholarship Fall 2015 INVESTIGATION OF AMBIENT SEISMIC NOISE USING SEISMIC INTERFEROMETRY IN WESTERN MONTANA Natalia Krzywosz Montana Tech of the University of Montana Follow this and additional works at: http://digitalcommons.mtech.edu/grad_rsch Part of the Geophysics and Seismology Commons Recommended Citation Krzywosz, Natalia, "INVESTIGATION OF AMBIENT SEISMIC NOISE USING SEISMIC INTERFEROMETRY IN WESTERN MONTANA" (2015). Graduate Theses & Non-Theses. 57. http://digitalcommons.mtech.edu/grad_rsch/57 This Thesis is brought to you for free and open access by the Student Scholarship at Digital Commons @ Montana Tech. It has been accepted for inclusion in Graduate Theses & Non-Theses by an authorized administrator of Digital Commons @ Montana Tech. For more information, please contact [email protected]. INVESTIGATION OF AMBIENT SEISMIC NOISE USING SEISMIC INTERFEROMETRY IN WESTERN MONTANA by Natalia Krzywosz A thesis submitted in partial fulfillment of the requirements for the degree of Master of Science in Geophysical Engineering Montana Tech 2015 ii Abstract Passive seismic interferometry is a process by which ambient noise data recorded at different seismic stations can be cross-correlated to estimate Green's functions. In the past, both surface waves and body waves have successfully been extracted by cross-correlation of ambient noise data on both regional and global scales. In this study, I have generated Matlab code to simulate an application of seismic interferometry on a synthetic model with pre-defined layers and p-wave velocities. For areas with known velocity models, the Matlab code produced in this study can be used to generate synthetic seismograms, and model the effects of cross-correlation on receiver responses. -
Seismic Time-Lapse Interferometry Across Scales
CHAPTER TWO Seismic time-lapse interferometry across scales Anne Obermanna,*, Gregor Hillersb aSwiss Seismological Service, ETH, Zurich, Switzerland bInstitute of Seismology, University of Helsinki, Helsinki, Finland *Corresponding author: e-mail address: [email protected] Contents 1. Introduction 66 2. Basic principles of seismic interferometry 69 2.1 Reconstruction of the Green’s function 69 2.2 Requirements for time-lapse monitoring 73 3. Noise-based interferometry 74 3.1 Spatio-temporal distribution of seismic noise sources 74 3.2 Noise-based tomographic imaging 78 3.3 Noise-based monitoring 79 4. Reflected-wave interferometry 80 5. Coda-wave interferometry (CWI) 82 5.1 Seismic coda waves 82 5.2 Different propagation regimes 84 5.3 Characteristic lengths in multiply scattering media 86 6. Detecting and locating time-lapse Changes 88 6.1 Conceptual differences between time-lapse changes in velocity and amplitude 88 6.2 Detecting time-lapse changes 89 6.3 Spatial location of CWI time-lapse changes 96 7. Applications across scales 98 7.1 Nondestructive material testing using diffuse ultrasound 99 7.2 Passive elastography: Seismology of the human body using shear waves from muscle activities 102 7.3 Monitoring applications on the local scale (meter–kilometer) using anthropogenic and oceanic noise 106 7.4 Passive monitoring at the regional scale using the ambient seismic field 118 8. Outlook 126 Acknowledgment 127 References 127 # Advances in Geophysics, Volume 60 2019 Elsevier Inc. 65 ISSN 0065-2687 All rights reserved. https://doi.org/10.1016/bs.agph.2019.06.001 66 Anne Obermann and Gregor Hillers 1. -
Passive Seismic Interferometry for Subsurface Imaging
Encyclopedia of Earthquake Engineering DOI 10.1007/978-3-642-36197-5_378-1 # Springer-Verlag Berlin Heidelberg 2015 Passive Seismic Interferometry for Subsurface Imaging Deyan Draganova* and Elmer Ruigrokb aDepartment of Geoscience and Engineering, Delft University of Technology, Delft, The Netherlands bDepartment of Earth Sciences, Utrecht University, Utrecht, The Netherlands Synonyms Ambient noise; Body waves; Cross-correlation; Green’s function retrieval; Reflection imaging; Reflec- tions; Seismic interferometry; Teleseismic arrivals Introduction Seismic interferometry is a method that allows the retrieval of the seismic response at one receiver from a virtual source at the position of another receiver. The method was first proposed in the seismic exploration community by Claerbout (1968) for a horizontally layered subsurface. The author showed that by autocorrelating the transmission response recorded at a receiver at the Earth’s surface from noise sources in the subsurface, one would retrieve the reflection response at this receiver from a virtual source also at that location. In the following decades, the applications of this idea were rare (Scherbaum 1987; Duvall et al. 1993; Daneshvar et al. 1995). But from the turn of the century, the fortunes changed and the method rapidly gained in popularity. This happened in the seismological community, due to applications to earthquake coda waves (Campillo and Paul 2003) and ambient noise (Shapiro and Campillo 2004), and in the exploration seismic community, due to the development of a solid theory for 3D media (Wapenaar 2004) and proposal to use the method also with controlled sources (Schuster et al. 2004). Lately, the method of seismic interferometry has found different applications, like in imaging of the subsurface, monitoring of changes in the subsurface, quality-factor estimation, suppression of surface waves, etc., at different scales with different types of sources. -
Seismic Interferometry and Stationary Phase at Caustics
Originally published as: Snieder, R., Sens-Schönfelder, C. (2015): Seismic interferometry and stationary phase at caustics. - Journal of Geophysical Research, 120, 6, p. 4333-4343. DOI: http://doi.org/10.1002/2014JB011792 Journal of Geophysical Research: Solid Earth RESEARCH ARTICLE Seismic interferometry and stationary phase at caustics 10.1002/2014JB011792 Roel Snieder1,2 and Christoph Sens-Schönfelder1 Key Points: • Explain how seismic interferometry 1Deutsches GeoForschungsZentrum, Potsdam, Germany, 2Center for Wave Phenomena, Colorado School of Mines, extracts waveforms at caustics Golden, Colorado, USA • At caustics the stationary phase zones are very large • This explains observations of waveforms extracted at caustics Abstract Waves that propagate between two receivers can be extracted by cross correlating noise (e.g., antipodes) recorded at these receivers if the noise is generated by sources on a closed surface surrounding the receivers. This concept is called seismic interferometry. Of all these noise sources, those for whom the travel Correspondence to: time difference for propagation to the two receivers is in the stationary phase zone, give the dominant R. Snieder, contribution. In this paper we analyze the stationary phase properties when one receiver is at a caustic for [email protected] waves leaving the other receiver. A simple model for a waveguide and a general treatment of caustics show that, at a caustic, the curvature of the travel time difference at the stationary phase zone vanishes. As a result Citation: the stationary phase region is considerably wider at a caustic than at other points, and it is more likely that Snieder, R., and C. Sens-Schönfelder noise sources are present in the stationary phase region. -
The Virtual Seismometer Method: Its Theory, History, and Promise
EARTHQUAKE SEISMOLOGY FINAL PAPER 2017 1 The virtual seismometer method: Its theory, history, and promise Jens-Erik Lund Snee Abstract—The virtual seismometer method (VSM), also involves crosscorrelating the coda waves between pairs called inter-source seismic interferometry, is a technique of earthquakes recorded at a single station, repeating this for finding the Green function between nearby earthquakes by estimating the response that would be detected at one process for multiple stations, and then stacking the results earthquake location if it were a seismometer observing the to find the Green function between each earthquake pair. others. This promising new type of seismic interferometry This procedure can be repeated for any number of nearby has found diverse applications in the past several years, although applications are surprisingly few considering the earthquakes to estimate the path parameters between them, power of the technique. In this paper, I summarize the hence potentially populating the subsurface in an area history of seismic interferometry and also the specific case with this information, even if seismometers are sparse. of VSM. I provide the theoretical basis for both of these techniques, as well as several recent applications, most In this document, I present the history and theoretical notably for continued methods development in the field of background of this promising new technique, and then I seismic interferometry. This report is intended to highlight discuss its potential uses to advance topics of active ways -
Interferometric Surface-Wave Isolation and Removal
GEOPHYSICS, VOL.72, NO. 5 ͑SEPTEMBER-OCTOBER 2007͒; P.A69–A73, 4 FIGS. 10.1190/1.2761967 Interferometric surface-wave isolation and removal David F. Halliday1, Andrew Curtis2, Johan O. A. Robertsson3, and Dirk-Jan van Manen3 When using active sources, theory indicates that sources are re- ABSTRACT quired to form a surface that bounds the portion of the earth in which we are interested. In practice, this requirement can be relaxed. In the The removal of surface waves ͑ground roll͒ from land seis- virtual source method of Bakulin and Calvert ͑2004, 2006͒, for ex- mic data is critical in seismic processing because these waves ample, sources are located only at ͑or near͒ the earth’s surface and re- tend to mask informative body-wave arrivals. Removal be- ceivers are placed in a borehole. Recordings from these sources are comes difficult when surface waves are scattered, and data used to create virtual sources at the downhole receivers. quality is often impaired. We apply a method of seismic inter- In the related field of passive seismic interferometry, crosscorrela- ferometry, using both sources and receivers at the surface, to tions of ambient noise at periods of 5–20 s can produce estimates of estimate the surface-wave component of the Green’s function the ͑direct͒ Rayleigh wave between surface receivers ͑Shapiro and between any two points. These estimates are subtracted adap- Campillo, 2004; Shapiro et al., 2005͒. The authors argue that the tively from seismic survey data, providing a new method of Rayleigh-wave component is isolated in these crosscorrelations be- ground-roll removal that is not limited to nonscattering cause ͑a͒ Rayleigh waves dominate the Green’s function between regions. -
Tutorial on Seismic Interferometry: Part 2 — Underlying Theory and New Advances
GEOPHYSICS, VOL.75, NO. 5 ͑SEPTEMBER-OCTOBER 2010͒; P.75A211–75A227, 10 FIGS. 10.1190/1.3463440 Tutorial on seismic interferometry: Part 2 — Underlying theory and new advances Kees Wapenaar1, Evert Slob1, Roel Snieder2, and Andrew Curtis3 ABSTRACT ent sources, gives the Green’s function emitted by a virtual source at the position of one of the receivers and observed by the In the 1990s, the method of time-reversed acoustics was devel- other receiver. This Green’s function representation for seismic oped. This method exploits the fact that the acoustic wave equa- interferometry is based on the assumption that the medium is tion for a lossless medium is invariant for time reversal. When ul- lossless and nonmoving. Recent developments, circumventing trasonic responses recorded by piezoelectric transducers are re- these assumptions, include interferometric representations for versed in time and fed simultaneously as source signals to the attenuating and/or moving media, as well as unified representa- transducers, they focus at the position of the original source, even tions for waves and diffusion phenomena, bending waves, quan- when the medium is very complex. In seismic interferometry the tum mechanical scattering, potential fields, elastodynamic, elec- time-reversed responses are not physically sent into the earth, but tromagnetic, poroelastic, and electroseismic waves. Significant they are convolved with other measured responses. The effect is improvements in the quality of the retrieved Green’s functions essentially the same: The time-reversed signals focus and create have been obtained with interferometry by deconvolution. A a virtual source which radiates waves into the medium that are trace-by-trace deconvolution process compensates for complex subsequently recorded by receivers.