Impact Mechanics at Meteor Crater, Arizona
Total Page:16
File Type:pdf, Size:1020Kb
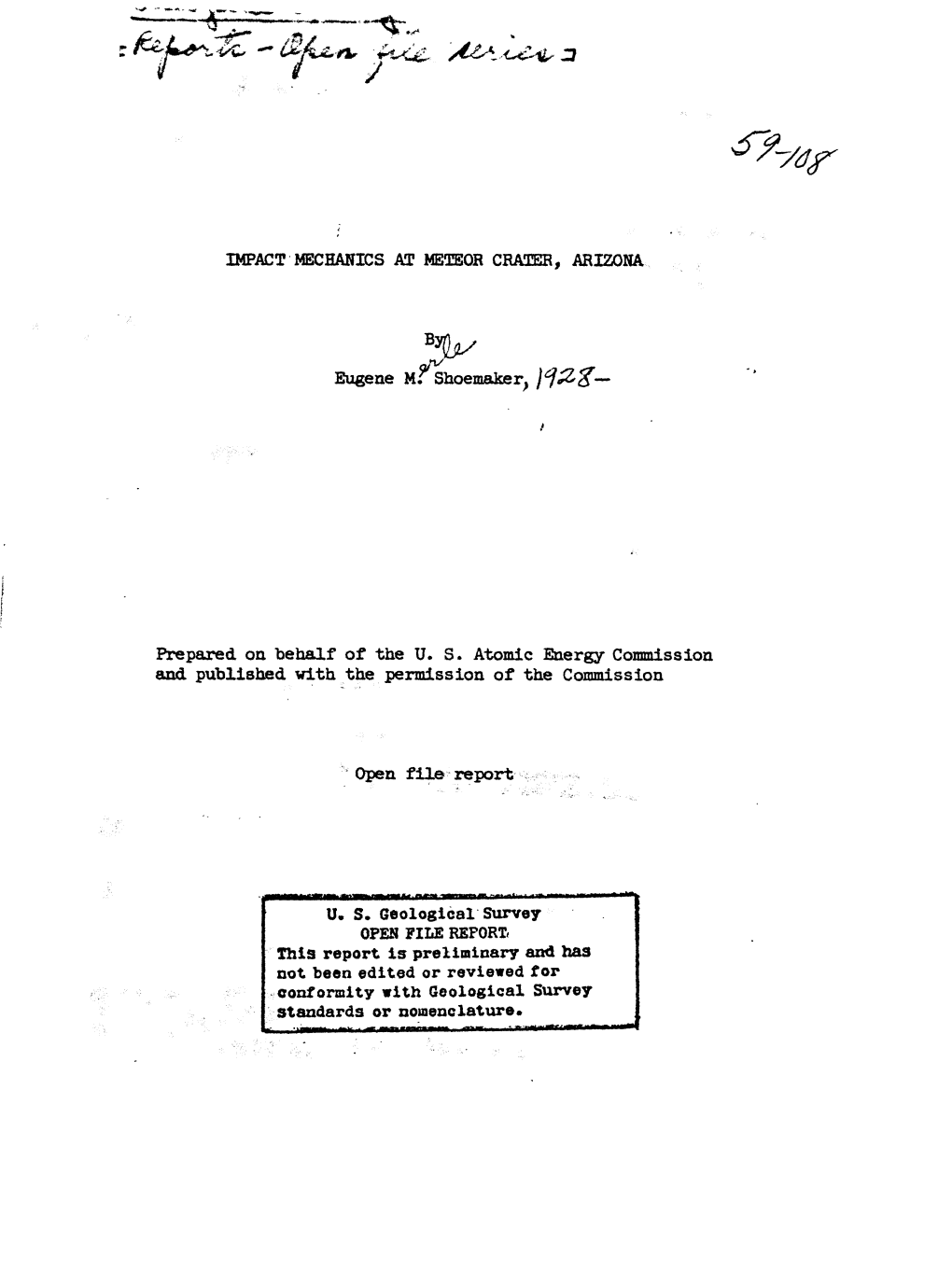
Load more
Recommended publications
-
10-1 CHAPTER 10 DEFORMATION 10.1 Stress-Strain Diagrams And
EN380 Naval Materials Science and Engineering Course Notes, U.S. Naval Academy CHAPTER 10 DEFORMATION 10.1 Stress-Strain Diagrams and Material Behavior 10.2 Material Characteristics 10.3 Elastic-Plastic Response of Metals 10.4 True stress and strain measures 10.5 Yielding of a Ductile Metal under a General Stress State - Mises Yield Condition. 10.6 Maximum shear stress condition 10.7 Creep Consider the bar in figure 1 subjected to a simple tension loading F. Figure 1: Bar in Tension Engineering Stress () is the quotient of load (F) and area (A). The units of stress are normally pounds per square inch (psi). = F A where: is the stress (psi) F is the force that is loading the object (lb) A is the cross sectional area of the object (in2) When stress is applied to a material, the material will deform. Elongation is defined as the difference between loaded and unloaded length ∆푙 = L - Lo where: ∆푙 is the elongation (ft) L is the loaded length of the cable (ft) Lo is the unloaded (original) length of the cable (ft) 10-1 EN380 Naval Materials Science and Engineering Course Notes, U.S. Naval Academy Strain is the concept used to compare the elongation of a material to its original, undeformed length. Strain () is the quotient of elongation (e) and original length (L0). Engineering Strain has no units but is often given the units of in/in or ft/ft. ∆푙 휀 = 퐿 where: is the strain in the cable (ft/ft) ∆푙 is the elongation (ft) Lo is the unloaded (original) length of the cable (ft) Example Find the strain in a 75 foot cable experiencing an elongation of one inch. -
Fstate Scientist: Omond Mckillop Solandt and Government Science
fState Scientist: Omond McKillop Solandt and Government Science in War and Hostile Peace, 1939-1956/ Scientifique.de l'Etat: Omond McKillop Solandt et la Science du Gouvernement lors de la Guerre et de la Paix Hostile, 1939-1956 A Thesis Submitted to the Division of Graduate Studies of the Royal Military College of Canada by Jason Sean Ridler, MA Royal Military College of Canada, 2001 BA (Hons.) York University, 1999 In Partial Fulfillment of the Requirements for the Degree of Doctor of Philosophy August 2008 ©This thesis may be used within the Department of National Defence but copyright for open publication remains the property of the author. Library and Bibliotheque et 1*1 Archives Canada Archives Canada Published Heritage Direction du Branch Patrimoine de I'edition 395 Wellington Street 395, rue Wellington Ottawa ON K1A0N4 Ottawa ON K1A0N4 Canada Canada Your file Votre reference ISBN: 978-0-494-47901-8 Our file Notre reference ISBN: 978-0-494-47901-8 NOTICE: AVIS: The author has granted a non L'auteur a accorde une licence non exclusive exclusive license allowing Library permettant a la Bibliotheque et Archives and Archives Canada to reproduce, Canada de reproduire, publier, archiver, publish, archive, preserve, conserve, sauvegarder, conserver, transmettre au public communicate to the public by par telecommunication ou par Plntemet, prefer, telecommunication or on the Internet, distribuer et vendre des theses partout dans loan, distribute and sell theses le monde, a des fins commerciales ou autres, worldwide, for commercial or non sur support microforme, papier, electronique commercial purposes, in microform, et/ou autres formats. paper, electronic and/or any other formats. -
Cross-References ASTEROID IMPACT Definition and Introduction History of Impact Cratering Studies
18 ASTEROID IMPACT Tedesco, E. F., Noah, P. V., Noah, M., and Price, S. D., 2002. The identification and confirmation of impact structures on supplemental IRAS minor planet survey. The Astronomical Earth were developed: (a) crater morphology, (b) geo- 123 – Journal, , 1056 1085. physical anomalies, (c) evidence for shock metamor- Tholen, D. J., and Barucci, M. A., 1989. Asteroid taxonomy. In Binzel, R. P., Gehrels, T., and Matthews, M. S. (eds.), phism, and (d) the presence of meteorites or geochemical Asteroids II. Tucson: University of Arizona Press, pp. 298–315. evidence for traces of the meteoritic projectile – of which Yeomans, D., and Baalke, R., 2009. Near Earth Object Program. only (c) and (d) can provide confirming evidence. Remote Available from World Wide Web: http://neo.jpl.nasa.gov/ sensing, including morphological observations, as well programs. as geophysical studies, cannot provide confirming evi- dence – which requires the study of actual rock samples. Cross-references Impacts influenced the geological and biological evolu- tion of our own planet; the best known example is the link Albedo between the 200-km-diameter Chicxulub impact structure Asteroid Impact Asteroid Impact Mitigation in Mexico and the Cretaceous-Tertiary boundary. Under- Asteroid Impact Prediction standing impact structures, their formation processes, Torino Scale and their consequences should be of interest not only to Earth and planetary scientists, but also to society in general. ASTEROID IMPACT History of impact cratering studies In the geological sciences, it has only recently been recog- Christian Koeberl nized how important the process of impact cratering is on Natural History Museum, Vienna, Austria a planetary scale. -
Geoscience and a Lunar Base
" t N_iSA Conference Pubhcatmn 3070 " i J Geoscience and a Lunar Base A Comprehensive Plan for Lunar Explora, tion unclas HI/VI 02907_4 at ,unar | !' / | .... ._-.;} / [ | -- --_,,,_-_ |,, |, • • |,_nrrr|l , .l -- - -- - ....... = F _: .......... s_ dd]T_- ! JL --_ - - _ '- "_r: °-__.......... / _r NASA Conference Publication 3070 Geoscience and a Lunar Base A Comprehensive Plan for Lunar Exploration Edited by G. Jeffrey Taylor Institute of Meteoritics University of New Mexico Albuquerque, New Mexico Paul D. Spudis U.S. Geological Survey Branch of Astrogeology Flagstaff, Arizona Proceedings of a workshop sponsored by the National Aeronautics and Space Administration, Washington, D.C., and held at the Lunar and Planetary Institute Houston, Texas August 25-26, 1988 IW_A National Aeronautics and Space Administration Office of Management Scientific and Technical Information Division 1990 PREFACE This report was produced at the request of Dr. Michael B. Duke, Director of the Solar System Exploration Division of the NASA Johnson Space Center. At a meeting of the Lunar and Planetary Sample Team (LAPST), Dr. Duke (at the time also Science Director of the Office of Exploration, NASA Headquarters) suggested that future lunar geoscience activities had not been planned systematically and that geoscience goals for the lunar base program were not articulated well. LAPST is a panel that advises NASA on lunar sample allocations and also serves as an advocate for lunar science within the planetary science community. LAPST took it upon itself to organize some formal geoscience planning for a lunar base by creating a document that outlines the types of missions and activities that are needed to understand the Moon and its geologic history. -
Qiao Et Al., Sosigenes Pit Crater Age 1/51
Qiao et al., Sosigenes pit crater age 1 2 The role of substrate characteristics in producing anomalously young crater 3 retention ages in volcanic deposits on the Moon: Morphology, topography, 4 sub-resolution roughness and mode of emplacement of the Sosigenes Lunar 5 Irregular Mare Patch (IMP) 6 7 Le QIAO1,2,*, James W. HEAD2, Long XIAO1, Lionel WILSON3, and Josef D. 8 DUFEK4 9 1Planetary Science Institute, School of Earth Sciences, China University of 10 Geosciences, Wuhan 430074, China. 11 2Department of Earth, Environmental and Planetary Sciences, Brown University, 12 Providence, RI 02912, USA. 13 3Lancaster Environment Centre, Lancaster University, Lancaster LA1 4YQ, UK. 14 4School of Earth and Atmospheric Sciences, Georgia Institute of Technology, Atlanta, 15 Georgia 30332, USA. 16 *Corresponding author E-mail: [email protected] 17 18 19 20 Key words: Lunar/Moon, Sosigenes, irregular mare patches, mare volcanism, 21 magmatic foam, lava lake, dike emplacement 22 23 24 25 1/51 Qiao et al., Sosigenes pit crater age 26 Abstract: Lunar Irregular Mare Patches (IMPs) are comprised of dozens of small, 27 distinctive and enigmatic lunar mare features. Characterized by their irregular shapes, 28 well-preserved state of relief, apparent optical immaturity and few superposed impact 29 craters, IMPs are interpreted to have been formed or modified geologically very 30 recently (<~100 Ma; Braden et al. 2014). However, their apparent relatively recent 31 formation/modification dates and emplacement mechanisms are debated. We focus in 32 detail on one of the major IMPs, Sosigenes, located in western Mare Tranquillitatis, 33 and dated by Braden et al. -
Impact of Flow Velocity on Surface Particulate Fouling - Theoretical Approach
Journal of American Science 2012;8(9) http://www.jofamericanscience.org Impact of Flow velocity on Surface Particulate Fouling - Theoretical Approach Mostafa M. Awad Mech. Power Eng. Dept., Faculty of Engineering, Mansoura University, Egypt [email protected] Abstract: The objective of this research is to study the effect of flow velocity on surface fouling. A new theoretical approach showing the effect of flow velocity on the particulate fouling has been developed. This approach is based on the basic fouling deposition and removal processes. The present results show that, the flow velocity has a strong effect on both the fouling rate and the asymptotic fouling factor; where the flow velocity affects both the deposition and removal processes. Increasing flow velocity results in decreasing both of the fouling rate and asymptotic values. Comparing the obtained theoretical results with available experimental ones showed good agreement between them. The developed model can be used as a very useful tool in the design and operation of the heat transfer equipment by controlling the parameters affecting fouling processes. [Mostafa M. Awad. Impact of Flow velocity on Surface Particulate Fouling - Theoretical Approach. J Am Sci 2012;8(9):442-449]. (ISSN: 1545-1003). http://www.jofamericanscience.org. 62 Keywords: Surface fouling, flow velocity, particle sticking, mass transfer. 1. Introduction increasing the flow velocity. They also found that the Fouling of heat transfer surface is defined as the asymptotic fouling factor is very sensitive to the flow accumulation of unwanted material on the heat transfer velocity especially at low flow velocities where this surface. This accumulation deteriorates the ability of sensitivity decreases with increasing flow velocity. -
Glossary Glossary
Glossary Glossary Albedo A measure of an object’s reflectivity. A pure white reflecting surface has an albedo of 1.0 (100%). A pitch-black, nonreflecting surface has an albedo of 0.0. The Moon is a fairly dark object with a combined albedo of 0.07 (reflecting 7% of the sunlight that falls upon it). The albedo range of the lunar maria is between 0.05 and 0.08. The brighter highlands have an albedo range from 0.09 to 0.15. Anorthosite Rocks rich in the mineral feldspar, making up much of the Moon’s bright highland regions. Aperture The diameter of a telescope’s objective lens or primary mirror. Apogee The point in the Moon’s orbit where it is furthest from the Earth. At apogee, the Moon can reach a maximum distance of 406,700 km from the Earth. Apollo The manned lunar program of the United States. Between July 1969 and December 1972, six Apollo missions landed on the Moon, allowing a total of 12 astronauts to explore its surface. Asteroid A minor planet. A large solid body of rock in orbit around the Sun. Banded crater A crater that displays dusky linear tracts on its inner walls and/or floor. 250 Basalt A dark, fine-grained volcanic rock, low in silicon, with a low viscosity. Basaltic material fills many of the Moon’s major basins, especially on the near side. Glossary Basin A very large circular impact structure (usually comprising multiple concentric rings) that usually displays some degree of flooding with lava. The largest and most conspicuous lava- flooded basins on the Moon are found on the near side, and most are filled to their outer edges with mare basalts. -
Planetary Surfaces
Chapter 4 PLANETARY SURFACES 4.1 The Absence of Bedrock A striking and obvious observation is that at full Moon, the lunar surface is bright from limb to limb, with only limited darkening toward the edges. Since this effect is not consistent with the intensity of light reflected from a smooth sphere, pre-Apollo observers concluded that the upper surface was porous on a centimeter scale and had the properties of dust. The thickness of the dust layer was a critical question for landing on the surface. The general view was that a layer a few meters thick of rubble and dust from the meteorite bombardment covered the surface. Alternative views called for kilometer thicknesses of fine dust, filling the maria. The unmanned missions, notably Surveyor, resolved questions about the nature and bearing strength of the surface. However, a somewhat surprising feature of the lunar surface was the completeness of the mantle or blanket of debris. Bedrock exposures are extremely rare, the occurrence in the wall of Hadley Rille (Fig. 6.6) being the only one which was observed closely during the Apollo missions. Fragments of rock excavated during meteorite impact are, of course, common, and provided both samples and evidence of co,mpetent rock layers at shallow levels in the mare basins. Freshly exposed surface material (e.g., bright rays from craters such as Tycho) darken with time due mainly to the production of glass during micro- meteorite impacts. Since some magnetic anomalies correlate with unusually bright regions, the solar wind bombardment (which is strongly deflected by the magnetic anomalies) may also be responsible for darkening the surface [I]. -
Technical Note
NASA TN D-1493 O5 C,/ 1-4 I Q LOAJN' ? < TECHNICAL NOTE D-1493 ENVIRONMENTAL PROBLEMS OF SPACE FLIGHT STRUCTURES H. METEOROID HAZARD Prepared by John R. Davidson and Paul E. Sandorff in collaboration with the NASA Research Advisory Committee on Missile and Space Vehicle Structures Langley Research Center Langley Station, Hampton, Va. NATIONAL AERONAUTICS AND SPACE ADMINISTRATION WASHINGTON January 1963 TECH LIBRARY KAFB, NM Illllllll TABLE OF CONTENTS 0153^57 Page PREFACE li MEMBERS OF THE NASA RESEARCH ADVISORY COMMITTEE ON MISSILE AND SPACE VEHICLE STRUCTURES in SUMMARY 1 INTRODUCTION 1 SYMBOLS -. 2 METEOROID ENVIRONMENT ^ Asteroidal Particles ; . k Cometary Particles 5 Tektites 8 Pertubation Forces 9 Observations of Meteors From the Earth 10 Satellites and Space Probes 15 Research in Progress 18 INTERACTION BETWEEN METEOROID ENVIRONMENT AND STRUCTURE 19 Historical Aspects of the Plate Perforation Problem 19 Physical Description of High-Speed Impact Phenomena 20 Modern Theories of Penetration 22 Experimental Data .__ 26 PROTECTION METHODS 30 Types of Structural Damage 30 Application of Meteoroid Flux Data and Impact Data to Design Problems 30 RESEARCH IN PROGRESS 36 Ground Research 36 Flight Test Research 36 RECOMMENDED METEOROID RESEARCH PROGRAM 36 REFERENCES kO BIBLIOGRAPHY 1*7 TABLES 49 FIGURES 61 PREFACE The exploration, study, and definition of the environmental conditions which exist in space beyond the earth's atmosphere form an essential part of the current space-age effort. The development of this science is of especial interest to the vehicle structures designer, who must make decisions today that will establish the capability and efficiency of the space hardware in use 5 °r 10 years hence. -
Destination Moon
Ill ".=\'.\, . : t.\ i...ii. 4'i.' --- v 1, 2*..- e> . 1.... ...*f. - .. .. .../C-'.»i.:5.1:• \ I: .. ...: ... ':4r-... - i.. ·.· I . I ·#. - I, :P.k 0 1 '. -1-3:Z:,ile<52.4 -2. .3••••4 - ....., 1 - I ..... 3/4 I <:.9, . /•/f••94*/ ,,iS· .... I ... ....... 9492./i- ..·,61« 4/.th_ r-*- .. -I,r * 116.-452*:••:il-.--•• . .....a• 1 --, •.11:E I . ".59/.-R:&6.Aidillizatu:ill'Illrclilllll 9 St" "' 4.... ... 'Ablf*liE•*.0/8/49/18•09#J'£• .-t-i «, ...' '-t ---- ·· -.t-:.:.'• --,•1*4*14-=..»r,3.hk= 4-lk/ *7·· •-,·:s - '' 21:1 . -0.6..ts-9....4559*"4914*Em'6rt'" .. « 036 ·.. '.... ..'·2&4:i•#+,M/ri:.1*-%,TYRf/036B.e... - ....2:'. .. *... '. '!..i. '':3..i-,f....35"ti F:Y.'..t•: &.....:. 39•'.•........ - ..., ..7. .- P 036.1..' -.Zi - , 93327 . « tr. 1 '. .- I . '.. I ... .'... 'SS> <13· '9•25 ."'t-·,·:·- " :· •t :Ali.•'.'; • - 'r ...• r..... ' ... .... .. " .4 4 ..2- -.*.. 3. .. : , _sry»5433.5.,+· i<29*643, W5• ./'..'r· -. 4-··./.-i--fR...1-L...'.te....bkir.i<Ativi•haRN,1.254i'*"*61»4-=•• 6.... 61 . 31 /'- 5 301... ....•L . , . - - . '2.1 . ' -' »4 /2/323#9/44 ZEIII:ill)*lill//Il/6,1-'.cri:rre I. p ·: , 4 ·,.3*4,·to.4.-•44 4 .,I-tS••7•,<•.•·:L,4..'::....,i... ..I·."i271/Ve;*m#6:d 54.... .. -,•....- . I..,418'I.,4I.,•....6..#i r:'.,:•St»1.·:·'7· ···- . t'-.,jit- 1-6#.19.-'*-. ' -e - .c, .•.. C'!•-042,.-• .... -I ./- =r- . 2 2. -" ' .t. · '., -042,,. ' '/.9. •SS ..2.. 11•11•»..••...qwGILLET+*frs'.. :. " . .... I .- , .. - ....,.k.... -< 3.5*«· ·: . ' i.1., li-, f 20- PR :21 . 0• . c. .Fh: ---, 1.4':. '•,. -,-t.Z:ft,•• •'r,4.4, 4 r • . -
Topographic Power Spectra of Cratered Terrains: 10.1002/2014JE004746 Theory and Application to the Moon
JournalofGeophysicalResearch: Planets RESEARCH ARTICLE Topographic power spectra of cratered terrains: 10.1002/2014JE004746 Theory and application to the Moon Key Points: Margaret A. Rosenburg1, Oded Aharonson2, and Re’em Sari3 • Impact cratering produces characteristic variations in the 1Division of Geological and Planetary Sciences, California Institute of Technology, Pasadena, California, USA, 2Department topographic PSD of Earth and Planetary Sciences, Weizmann Institute of Technology, Rehovot, Israel, 3Racah Institute of Physics, Hebrew • The size-frequency distribution and shape of craters control University of Jerusalem, Jerusalem, Israel PSD variations • We investigate the topographic PSD on model terrains and Impact cratering produces characteristic variations in the topographic power spectral density lunar topography Abstract (PSD) of cratered terrains, which are controlled by the size-frequency distribution of craters and the spectral content (shape) of individual features. These variations are investigated here in two parallel Correspondence to: approaches. First, a cratered terrain model, based on Monte Carlo emplacement of craters and benchmarked M. A. Rosenburg, [email protected] by an analytical formulation of the one-dimensional PSD, is employed to generate topographic surfaces at a range of size-frequency power law exponents and shape dependencies. For self-similar craters, the slope of the PSD, , varies inversely with that of the production function, , leveling off to 0 at high (surface Citation: Rosenburg, M. A., O. Aharonson, topography dominated by the smallest craters) and maintaining a roughly constant value ( ∼ 2) at low and R. Sari (2015), Topographic (surface topography dominated by the largest craters). The effects of size-dependent shape parameters power spectra of cratered terrains: and various crater emplacement rules are also considered. -
Regional Oral History Off Ice University of California the Bancroft Library Berkeley, California
Regional Oral History Off ice University of California The Bancroft Library Berkeley, California Richard B. Gump COMPOSER, ARTIST, AND PRESIDENT OF GUMP'S, SAN FRANCISCO An Interview Conducted by Suzanne B. Riess in 1987 Copyright @ 1989 by The Regents of the University of California Since 1954 the Regional Oral History Office has been interviewing leading participants in or well-placed witnesses to major events in the development of Northern California, the West,and the Nation. Oral history is a modern research technique involving an interviewee and an informed interviewer in spontaneous conversation. The taped record is transcribed, lightly edited for continuity and clarity, and reviewed by the interviewee. The resulting manuscript is typed in final form, indexed, bound with photographs and illustrative materials, and placed in The Bancroft Library at the University of California, Berkeley, and other research collections for scholarly use. Because it is primary material, oral history is not intended to present the final, verified, or complete narrative of events. It is a spoken account, offered by the interviewee in response to questioning, and as such it is reflective, partisan, deeply involved, and irreplaceable. All uses of this manuscript are covered by a legal agreement between the University of California and Richard B. Gump dated 7 March 1988. The manuscript is thereby made available for research purposes. All literary rights in the manuscript, including the right to publish, are reserved to The Bancroft Library of the University of California, Berkeley. No part of the manuscript may be quoted for publication without the written permission of the Director of The Bancroft Library of the University of California, Berkeley.