Gabe on Emergence of 2Nd Law on Short Time Scales
Total Page:16
File Type:pdf, Size:1020Kb
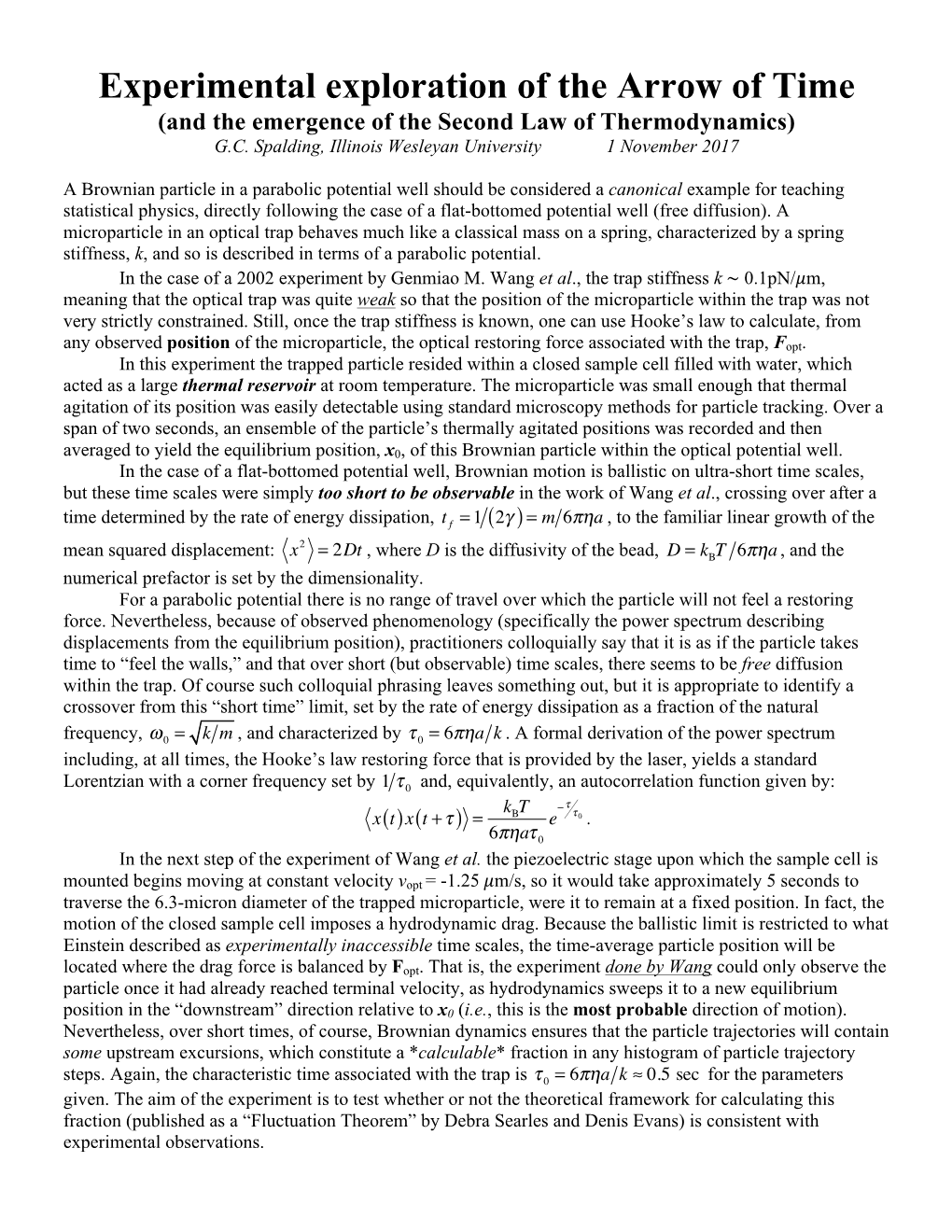
Load more
Recommended publications
-
How Nonequilibrium Thermodynamics Speaks to the Mystery of Life
CORE CONCEPTS How nonequilibrium thermodynamics speaks to the mystery of life CORE CONCEPTS Stephen Ornes, Science Writer In his 1944 book What is Life?, Austrian physicist Erwin Schrödinger argued that organisms stay alive pre- cisely by staving off equilibrium. “How does the living organism avoid decay?” he asks. “The obvious answer is: By eating, drinking, breathing and (in the case of plants) assimilating. The technical term is metabolism” (1). However, the second law of thermodynamics, and the tendency for an isolated system to increase in entropy, or disorder, comes into play. Schrödinger wrote that the very act of living is the perpetual effort to stave off disor- der for as long as we can manage; his examples show how living things do that at the macroscopic level by taking in free energy from the environment. For example, people release heat into their surroundings but avoid running out of energy by consuming food. The ultimate source of “negative entropy” on Earth, wrote Schrödinger, is the Sun. Recent studies suggest something similar is hap- pening at the microscopic level as well, as many cellular processes—ranging from gene transcription to intracellular transport—have underlying nonequi- librium drivers (2, 3). Indeed, physicists have found that nonequilibrium systems surround us. “Most of the world around us is in this situation,” says theoretical physicist Michael Cross at the California Institute of Technology, in Pasa- Attributes of living organisms, such as the flapping hair-like flagella of these single- dena. Cross is among many theorists who spent de- celled green algae known as Chlamydomonas, are providing insights into the cades chasing a general theory of nonequilibrium thermodynamics of nonequilibrium systems. -
Arxiv:1708.03422V2 [Cond-Mat.Stat-Mech]
Fluctuation Theorem and Central Limit Theorem for the Time-Reversible Nonequilibrium Baker Map William Graham Hoover Ruby Valley Research Institute Highway Contract 60, Box 601 Ruby Valley, NV 8983 (Dated: February 13, 2018) Abstract The nonequilibrium Time-Reversible Baker Map provides simple illustrations of the Fluctua- tion Theorem, the Central Limit Theorem, and the Biased Random Walk. This is material in preparation for the Book form of Carol’s and my 2016 Kharagpur Lectures. Comments welcome. Keywords: Fluctuation Theorem, Chaos, Lyapunov Exponents, Irreversibility, Second Law, Baker Map arXiv:1708.03422v2 [cond-mat.stat-mech] 14 Aug 2017 1 I. INTRODUCTION In 1993 Denis Evans, Eddie Cohen, and Gary Morriss discovered an interesting symmetry, by now “well-known”, in their studies of the periodic shear flow of 56 hard disks1. They kept track of the time-averaged Gibbs’ entropy changes associated with the flow as a function of the averaging time τ. At a strainrateǫ ˙ and over a time window τ the distribution of entropy 2 production rates approaches a smooth curve with a mean value, h (S/k˙ )=(V/T )ηǫ˙ iτ . The fluctuations about this mean necessarily satisfy the Central Limit Theorem for large τ. Evans, Cohen, and Morriss stressed that both positive and negative values of the entropy production can be observed if the system is not too small ( 56 soft disks in their case ) and τ is not too large ( a few collision times ). At equilibrium the positive and negative values even out over time. Away from equilibrium the positive values win out. The relatively few time intervals with negative values correspond to periods of entropy decrease. -
Arxiv:0805.1490V2 [Nlin.CD]
Simulation of Two- and Three-Dimensional Dense-Fluid Shear Flows via Nonequilibrium Molecular Dynamics. Comparison of Time-and-Space-Averaged Stresses from Homogeneous Doll’s and Sllod Shear Algorithms with those from Boundary-Driven Shear. Wm. G. Hoover and Carol G. Hoover Ruby Valley Research Institute Highway Contract 60, Box 598, Ruby Valley 89833, NV USA Janka Petravic Complex Systems in Biology Group Centre for Vascular Research The University of New South Wales Sydney NSW 2052, Australia (Dated: November 6, 2018) Abstract Homogeneous shear flows (with constant strainrate dvx/dy) are generated with the Doll’s and Sllod algorithms and compared to corresponding inhomogeneous boundary-driven flows. We use one-, two-, and three-dimensional smooth-particle weight functions for computing instantaneous spatial averages. The nonlinear normal stress differences are small, but significant, in both two and three space dimensions. In homogeneous systems the sign and magnitude of the shear- arXiv:0805.1490v2 [nlin.CD] 19 Jul 2008 plane stress difference, Pxx − Pyy, depend on both the thermostat type and the chosen shearflow algorithm. The Doll’s and Sllod algorithms predict opposite signs for this normal stress differ- ence, with the Sllod approach definitely wrong, but somewhat closer to the (boundary-driven) truth. Neither of the homogeneous shear algorithms predicts the correct ordering of the kinetic temperatures: Txx > Tzz > Tyy. PACS numbers: 02.70.Ns, 45.10.-b, 46.15.-x, 47.11.Mn, 83.10.Ff Keywords: Thermostats, Ergostats, Molecular Dynamics, Computational Methods, Smooth Particles 1 I. INTRODUCTION In the present work, we use nonequilibrium molecular dynamics[1] to study microscopic simulations of “simple shear flow” (also called “plane Couette flow”): vx ∝ y → Pxy ≡ Pyx ≡ −η[(∂vx/∂y)+(∂vy/∂x)] = −η(dvx/dy) = −ηǫ˙ . -
Classical and Quantum Out-Of-Equilibrium Dynamics
Classical and quantum out-of-equilibrium dynamics. Formalism and applications. Camille Aron To cite this version: Camille Aron. Classical and quantum out-of-equilibrium dynamics. Formalism and applications.. Data Analysis, Statistics and Probability [physics.data-an]. Université Pierre et Marie Curie - Paris VI, 2010. English. tel-00538099 HAL Id: tel-00538099 https://tel.archives-ouvertes.fr/tel-00538099 Submitted on 21 Nov 2010 HAL is a multi-disciplinary open access L’archive ouverte pluridisciplinaire HAL, est archive for the deposit and dissemination of sci- destinée au dépôt et à la diffusion de documents entific research documents, whether they are pub- scientifiques de niveau recherche, publiés ou non, lished or not. The documents may come from émanant des établissements d’enseignement et de teaching and research institutions in France or recherche français ou étrangers, des laboratoires abroad, or from public or private research centers. publics ou privés. THESE` DE DOCTORAT DE L’UNIVERSITE´ PIERRE ET MARIE CURIE Specialit´ e´ : Physique theorique´ Ecole´ doctorale : ≪ La physique, de la particule au solide ≫ Realis´ ee´ au Laboratoire de Physique Theorique´ et Hautes Energies´ Present´ ee´ par M. Camille ARON Pour obtenir le grade de Docteur de l’Universite´ Pierre et Marie Curie Dynamique hors d’equilibre´ classique et quantique. Formalisme et applications. Soutenue le 20 septembre 2010 devant le jury compose´ de M. Denis BERNARD (President´ du jury) M. Federico CORBERI (Examinateur) me M Leticia CUGLIANDOLO (Directrice de these)` M. Gabriel KOTLIAR (Rapporteur) M. Marco PICCO (Invite)´ M. Fred´ eric´ Van WIJLAND (Rapporteur) A bracadabra. REMERCIEMENTS ES tous premiers vont bien naturellement a` ma directrice de these,` Leticia Cugliandolo. -
On the Fluctuations of Dissipation: an Annotated Bibliography∗
Notes on the Dynamics of Disorder On the Fluctuations of Dissipation: An Annotated Bibliography∗ Tech. Note 005 (2019-07-28) http://threeplusone.com/ftbib Gavin E. Crooks 2010-2019 Origins: Fluctuation theorem: Evans et al. (1993) [39]. Foundations – Microscopic reversibility and detailed Evans-Searles fluctuation theorem: Evans and Searles balance: Origins: Tolman (1924) [11], Dirac (1924) [12], (1994) [41]. Gallavotti-Cohen fluctuation theorem: Tolman (1925) [14], Lewis (1925) [13], Fowler and Milne Gallavotti and Cohen (1995) [43]. Fluctuation theorem, (1925) [15]. Discussion: Onsager (1931) [18], Tolman first use of terminology: Gallavotti and Cohen (1995) [44]. (1938) [20], Crooks (1998) [57], Crooks (2011) [402]. Jarzynski equality: Jarzynski (1997) [50]. Crooks fluc- tuation theorem: Crooks (1999) [75]. Hatano-Sasa Experiments: Single molecule Jarzynski: Liphardt et al. fluctuation theorem: Hatano and Sasa (2001) [99]. Feed- (2002) [113]; single molecule fluctuation theorems: Collin back Jarzynski equality: Sagawa and Ueda (2010) [371]. et al. (2005) [209], Ritort (2006) [249]; dragged optically trapped colloid particle: Wang et al. (2002) [114], Wang et al. (2005) [190], Wang et al. (2005) [215]; Hatano and Reviews and expositions: For a gentle, modern in- Sasa with optically trapped colloid particle: Trepagnier troduction see Spinney and Ford (2013) [458], and et al. (2004) [165]; optically trapped colloid particle, time then try reading some of the other reviews in the varying spring constant: Carberry et al. (2004) [144], Car- same volume: Sagawa and Ueda (2013) [457], Reid berry et al. (2004) [166]; colloidal particle in periodic po- et al. (2013) [456], Gaspard (2013) [453]. See also tential: Speck et al. (2007) [294]; colloidal particle in vis- Evans and Searles (2002) [118], Harris and Schutz¨ coelastic media: Carberry et al. -
Ccp5 Programme-Latest
1 Advances in Theory and Simulation of non-Equilibrium Systems Joint CCP5-RSC Workshop: Advances in Theory and Simulation of non-Equilibrium Systems Imperial College London 26th June – 27th June, 2013 Statistical Mechanics & Thermodynamics Group 2 Advances in Theory and Simulation of non-Equilibrium Systems Joint CCP5-RSC Workshop: Advances in Theory and Simulation of non-Equilibrium Systems Imperial College London 26th June – 27th June, 2013 Dear Delegate, Non-equilibrium phenomena are ubiquitous in both the natural world and within the domain of applied science and engineering. Advances in understanding the non-equilibrium response of materials to changes in the mechanical and thermodynamic fields, including thermal, electric, magnetic and pressure gradients, is of significant pedagogical and practical importance, but also very challenging. Materials design and processing, energy recovery and energy conversion are just a few examples of obvious industrial relevance. Significant developments in statistical mechanics, most notably the Mayers' contribution to the virial equation of state and Green and Kubo's (linear response theory), coupled with the rapid development of simple, deterministic algorithms for simulating non-equilibrium systems, has opened the door to a tractable non-equilibrium thermodynamic theory applicable beyond the linear regime. Simulation is at its most useful and powerful when providing pseudo-experimental data to test these new theories. Atomistic simulation, for example is more usefully employed in suggesting new constitutive equations; recent work has suggested ways in which to handle the treatment of shockwaves and viscous flow in ultra thin films by going beyond Newton's law of viscosity and Fourier's law of heat conduction. -
Inventing a New America Through Discovery and Innovation in Science, Engineering, and Medicine
Research Directions Workshop Sponsored by the National Science Foundation (NSF). A related WTEC international study provided background for this workshop. That project was sponsored by NSF, the Department of Energy (DOE), the National Aeronautics and Space Administration (NASA), the National Institute for Biomedical Imaging and Bioengineering (NIBIB) and the National Library of Medicine (NLM) of the National Institutes of Health (NIH), the National Institute of Standards and Technology (NIST), and the Department of Defense (DOD). Chair Co-Chair Peter T. Cummings, Ph.D Sharon C. Glotzer, Ph.D. Vanderbilt University University of Michigan 303 Olin Hall 3074 H.H. Dow Building VU Station B 351604 Ann Arbor, MI 48109-2136 Nashville, TN 37235 [email protected] [email protected] This document is sponsored by the National Science Foundation (NSF) under grant No. ENG- 0925098 to the World Technology Evaluation Center, Inc. (WTEC). The Government has certain rights in this material. Any opinions, findings, and conclusions or recommendations expressed in this material are those of the authors and do not necessarily reflect the views of the United States Government, the authors’ parent institutions, or WTEC. Copyright 2010 by WTEC. The U.S. Government retains a nonexclusive and nontransferable license to exercise all exclusive rights provided by copyright. Full text of all WTEC reports is available at http://wtec.org. A sample of WTEC reports and information on obtaining them are on the inside back cover of this report. WTEC Mission WTEC specializes in assessments of international research and development in selected technologies under awards from the National Science Foundation (NSF), the Office of Naval Research (ONR), and other agencies. -
Statistical Mechanics of Nonequilibrium Liquids
statistical mechanics of nonequilibrium liquids statistical mechanics of nonequilibrium liquids Denis J. evans | Gary P. Morriss Published by ANU E Press The Australian National University Canberra ACT 0200, Australia Email: [email protected] This title is also available online at: http://epress.anu.edu.au/sm_citation.html Previously published by Academic Press Limited National Library of Australia Cataloguing-in-Publication entry Evans, Denis J. Statistical mechanics of nonequilibrium liquids. 2nd ed. Includes index. ISBN 9781921313226 (pbk.) ISBN 9781921313233 (online) 1. Statistical mechanics. 2. Kinetic theory of liquids. I. Morriss, Gary P. (Gary Phillip). II. Title. 530.13 All rights reserved. No part of this publication may be reproduced, stored in a retrieval system or transmitted in any form or by any means, electronic, mechanical, photocopying or otherwise, without the prior permission of the publisher. Cover design by Teresa Prowse Printed by University Printing Services, ANU First edition © 1990 Academic Press Limited This edition © 2007 ANU E Press Contents Preface ..................................................................................................... ix Biographies ............................................................................................... xi List of Symbols ....................................................................................... xiii 1. Introduction ......................................................................................... 1 References ....................................................................................... -
Nonequilibrium Molecular Dynamics Billy D
Cambridge University Press 978-0-521-19009-1 — Nonequilibrium Molecular Dynamics Billy D. Todd , Peter J. Daivis Frontmatter More Information Nonequilibrium Molecular Dynamics Theory, Algorithms and Applications This book describes the growing field of nonequilibrium molecular dynamics (NEMD), written in a form that will appeal to the general practitioner in molecular simulation. It introduces the theory fundamental to the field, namely nonequilibrium statistical mechanics and nonequilibrium thermodynamics, provides state-of-the-art algorithms and advice for designing reliable NEMD code, and examines applications for both atomic and molecular fluids. It discusses homogenous and inhomogeneous flows and pays considerable attention to studies of highly confined fluids. In addition to statistical mechanics and thermodynamics, the book covers such themes as temperature, thermo- dynamic fluxes and their computation, the theory and algorithms for homogeneous shear and elongational flows, response theory and its applications, heat and mass transport algorithms, applications in molecular rheology, highly confined fluids (nanofluidics), the phenomenon of slip and generalised hydrodynamics. Billy D. Todd undertook his bachelor and doctoral studies in physics at the University of Western Australia and Murdoch University in Perth, Australia. He then completed postdoctoral appointments at the University of Cambridge and the Australian National University, before moving to CSIRO in Melbourne in 1996. In 1999 he took up an aca- demic appointment at Swinburne University of Technology, where he is currently Pro- fessor and Chair of the Department of Mathematics. His research focus is on statistical mechanics, nonequilibrium molecular dynamics and computational nanofluidics. He is a Fellow of the Australian Institute of Physics and a former President of the Australian Society of Rheology. -
Liquid State Chemical Physics Professor Denis Evans Our Research Interests Include Nonequilibrium Statistical Mechanics and Thermodynamics
Liquid State Chemical Physics Professor Denis Evans Our research interests include nonequilibrium statistical mechanics and thermodynamics. We have been involved in the development of nearly all of the computer simulation algorithms used for the calculation of transport properties of classical atomic, molecular and short-chain polymeric fluids and lubricants. Algorithms that we have proposed are used to compute the viscosities, thermal conductivities, and diffusion coefficients for molecular fluids and fluid mixtures. These practical applications are based on the theory of nonequilibrium steady states, also developed by our group. Our theory of such systems provides a framework within which exact relationships between nonequilibrium fluctuations and measurable thermophysical properties have been proved. We derived the first exact, practical link between the theory of chaos, dynamical systems theory, and thermophysical properties. This link shows that a transport coefficient, like shear viscosity, is related in a direct, quantitative way to the stability of molecular trajectories. Later we derived the so-called Fluctuation Theorem (FT). This remarkable theorem gives an analytic expression for the probability that in a nonequilibrium steady state of finite size, observed for a finite time, the dissipative flux flows in the reverse direction to that required by the Second Law of Thermodynamics. Close to equilibrium the FT can be used to derive both Einstein and Green–Kubo relations for transport coefficients. In collaboration with members of the Polymers and Soft Condensed Matter Group, the FT has been verified experimentally. Fluctuation Theorem (FT) Work has continued on our proof that the so-called Gallavotti-Cohen FT (which only applies to steady states), in fact only applies to constant energy steady states. -
Edited Transcript of Interview with Michael Barber
CSIRO Oral History Collection Edited transcript of interview with Michael Barber Date of interview: 27th October 2017 Location: Swinburne University of Technology (Melbourne, Victoria) Interviewers: Tom Spurling and Terry Healy Copyright owned by Swinburne University of Technology and CSIRO. This transcript is licensed under a Creative Commons Attribution-NonCommercial-NoDerivs License. http://creativecommons.org/licenses/by-nc-nd/4.0/ 1 Swinburne University of Technology | CRICOS Provider 00111D | swinburne.edu.au Emeritus Professor Michael Newton Barber AO, BSc (Hons) (UNSW), PhD (Cornell), FAA, FTSE, FAICD Summary of interview Michael Barber was born in Sydney on 30 April 1947. In the early part of the interview, Michael talks about his experiences growing up in an academic household in Sydney and Hobart. He is a second generation university educated Australian. He recalls some of the visitors to his parent’s home, including Dr Jerry Price, a future Chairman of CSIRO. He also talks about his early interest in science and his mention in a 1965 ‘Heredity’ paper by his father on selection in natural populations. Michael attended Clarence High School in Hobart where the School Principal, Ed Smith, was also his science teacher. Michael remembers the long-term value of Mr Smith’s insistence good English expression in science essays. There ensues a detailed discussion about Michael’s mathematical education, including the influences of his key mentors. Michael describes his experiences as an academic at UNSW and the ANU, the effect of the Dawkins’ reforms and his growing interest in leadership roles. He talks about his recruitment to the University of Western Australia, and his contribution to the development of technology transfer policies there. -
Annual Report 2012
AIBN ANNUAL REPORT 2012 1 Vice-Chancellor’s message 63 International companies on board with 3 Director’s message bio jet fuel research 4 New board to raise AIBN’s 64 Functions showcase research and international profile industry collaborations 5 Leadership 65 Building links with industry 6 Our vision 66 AIBN start-up companies 7 2012 Highlights 67 International collaborations 8 Strategies aim to combat cell 68 International collaborations transformations 69 Computational modelling at the core 11 Research to keep aircraft flying of global networking 12 Tissue regeneration a step closer 70 Stem cell researchers benefit from 15 Microscopy helps art conservators global network preserve valuable artworks 71 AIBN gains Hendra funding 16 Key collaborations drive research 72 Leading academics gather at outcomes conference 18 Major successes in bioplastic research 73 International materials scientists share 21 Research and commercialisation push their research for needle-free vaccination 74 Engaging with international 23 Trojan horse approach targets researchers cancer cells 75 Facilities and research 24 Tenacious plastics become reality environment 27 Advancing understanding and 76 Infrastructure facilitates research exploitation of biological interactions 78 Enabling cutting-edge research with 28 Developing novel polymer protein expertise architectures for targeted applications 79 Funding secured for specialist facility 31 Nanomac has change of leadership 80 Microscopy and microanalysis 34 Trash to treasure 36 Personalising breast cancer treatment