Mberger Phd Thesis.Pdf
Total Page:16
File Type:pdf, Size:1020Kb
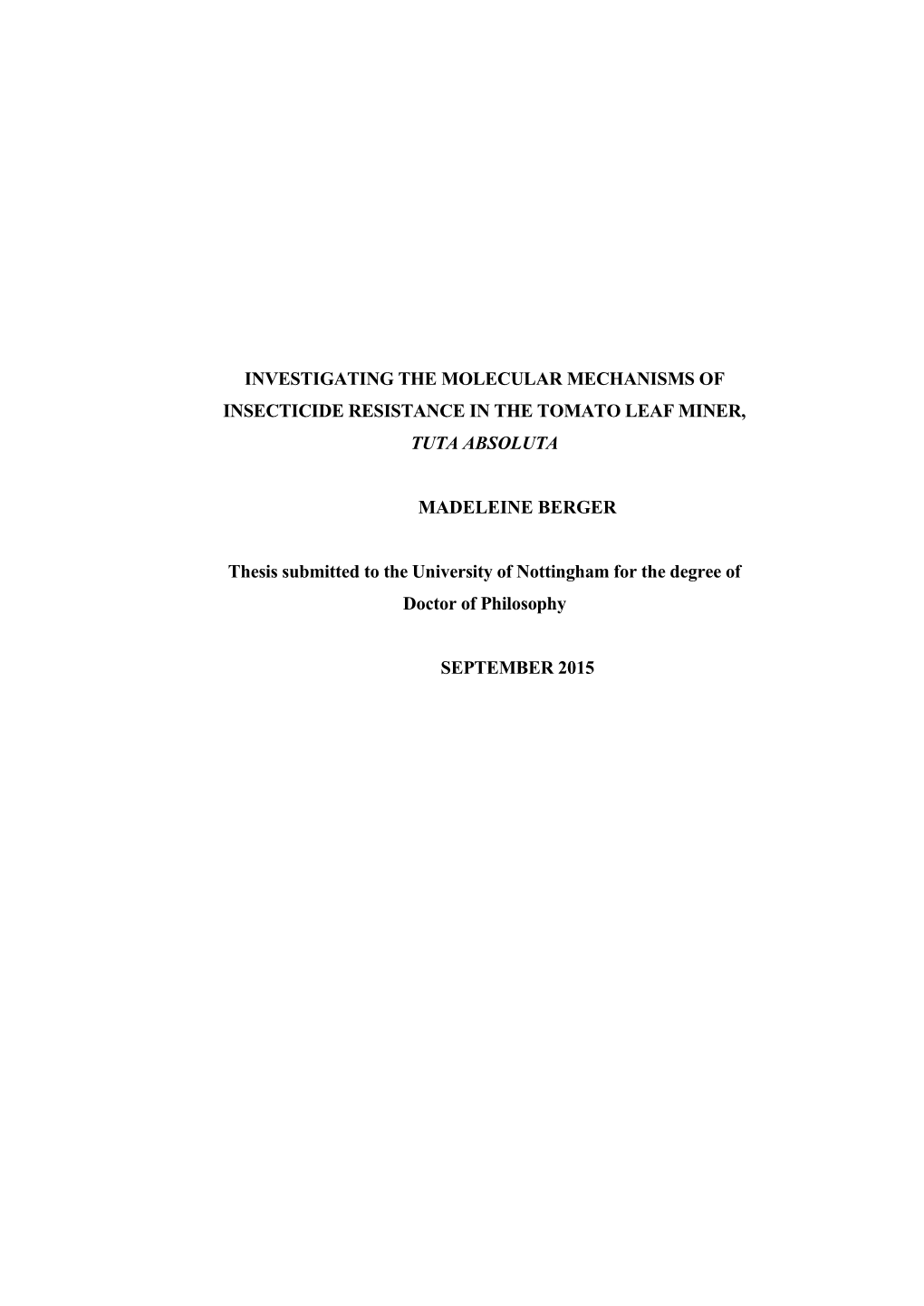
Load more
Recommended publications
-
Persistence of the Exotic Mirid Nesidiocoris Tenuis (Hemiptera: Miridae) in South Texas
insects Article Persistence of the Exotic Mirid Nesidiocoris tenuis (Hemiptera: Miridae) in South Texas Gabriela Esparza-Diaz 1,*, Thiago Marconi 1 , Carlos A. Avila 1,2 and Raul T. Villanueva 3,* 1 Texas A&M AgriLife Research, 2415 East Highway 83, Weslaco, TX 78596, USA; [email protected] (T.M.); [email protected] (C.A.A.) 2 Department of Horticultural Sciences, Texas A&M University, College Station, TX 77843, USA 3 University of Kentucky Research & Education Center, Department of Entomology, 348 University Drive, Princeton, KY 42445, USA * Correspondence: [email protected] (G.E.-D.); [email protected] (R.T.V.); Tel.: +1-(956)-9987281 (G.E.-D.); +1-(270)-365-7541-x21335 (R.T.V.) Simple Summary: The Rio Grande Valley is one of the most productive agricultural areas in the U.S, located in the southernmost part of Texas. In October 2013, we detected an exotic species of plant bug occurring in this region. It was identified as Nesidiocoris tenuis, which had a phytophagous behavior on tomato plants in the absence of its preferred prey. We confirmed the species with morphological and genetic tests. We monitored populations of N. tenuis in its introduction phase in commercial fields and corroborated its establishment in research fields for three consecutive years. The presence of N. tenuis could establish a new relationship of trophic insects to produce vegetables in the Rio Grande Valley. However, it is unknown whether the presence of N. tenuis in the Rio Grande Valley can help control pests of economic importance, such as whiteflies in cotton, or become a pest on sesame, which is an emerging crop in this region. -
Recherche D'alternatives Aux Pesticides Chimiques Pour Lutter Contre Tuta Absoluta
https://lib.uliege.be https://matheo.uliege.be Recherche d'alternatives aux pesticides chimiques pour lutter contre Tuta Absoluta (Meyrick, 1971) et de stratégies d'optimisation de l'utilisation de Nesidiocoris Tenuis (Reuter, 1895), prédateur de ce ravageurau Burkina Faso Auteur : Ouedraogo, Dominique Promoteur(s) : Verheggen, François Faculté : Gembloux Agro-Bio Tech (GxABT) Diplôme : Master de spécialisation en production intégrée et préservation des ressources naturelles en milieu urbain et péri-urbain Année académique : 2019-2020 URI/URL : http://hdl.handle.net/2268.2/10899 Avertissement à l'attention des usagers : Tous les documents placés en accès ouvert sur le site le site MatheO sont protégés par le droit d'auteur. Conformément aux principes énoncés par la "Budapest Open Access Initiative"(BOAI, 2002), l'utilisateur du site peut lire, télécharger, copier, transmettre, imprimer, chercher ou faire un lien vers le texte intégral de ces documents, les disséquer pour les indexer, s'en servir de données pour un logiciel, ou s'en servir à toute autre fin légale (ou prévue par la réglementation relative au droit d'auteur). Toute utilisation du document à des fins commerciales est strictement interdite. Par ailleurs, l'utilisateur s'engage à respecter les droits moraux de l'auteur, principalement le droit à l'intégrité de l'oeuvre et le droit de paternité et ce dans toute utilisation que l'utilisateur entreprend. Ainsi, à titre d'exemple, lorsqu'il reproduira un document par extrait ou dans son intégralité, l'utilisateur citera de manière complète les sources telles que mentionnées ci-dessus. Toute utilisation non explicitement autorisée ci-avant (telle que par exemple, la modification du document ou son résumé) nécessite l'autorisation préalable et expresse des auteurs ou de leurs ayants droit. -
Supplemental Information
Supplemental information Dissection of the genomic structure of the miR-183/96/182 gene. Previously, we showed that the miR-183/96/182 cluster is an intergenic miRNA cluster, located in a ~60-kb interval between the genes encoding nuclear respiratory factor-1 (Nrf1) and ubiquitin-conjugating enzyme E2H (Ube2h) on mouse chr6qA3.3 (1). To start to uncover the genomic structure of the miR- 183/96/182 gene, we first studied genomic features around miR-183/96/182 in the UCSC genome browser (http://genome.UCSC.edu/), and identified two CpG islands 3.4-6.5 kb 5’ of pre-miR-183, the most 5’ miRNA of the cluster (Fig. 1A; Fig. S1 and Seq. S1). A cDNA clone, AK044220, located at 3.2-4.6 kb 5’ to pre-miR-183, encompasses the second CpG island (Fig. 1A; Fig. S1). We hypothesized that this cDNA clone was derived from 5’ exon(s) of the primary transcript of the miR-183/96/182 gene, as CpG islands are often associated with promoters (2). Supporting this hypothesis, multiple expressed sequences detected by gene-trap clones, including clone D016D06 (3, 4), were co-localized with the cDNA clone AK044220 (Fig. 1A; Fig. S1). Clone D016D06, deposited by the German GeneTrap Consortium (GGTC) (http://tikus.gsf.de) (3, 4), was derived from insertion of a retroviral construct, rFlpROSAβgeo in 129S2 ES cells (Fig. 1A and C). The rFlpROSAβgeo construct carries a promoterless reporter gene, the β−geo cassette - an in-frame fusion of the β-galactosidase and neomycin resistance (Neor) gene (5), with a splicing acceptor (SA) immediately upstream, and a polyA signal downstream of the β−geo cassette (Fig. -
9-Azido Analogs of Three Sialic Acid Forms for Metabolic Remodeling Of
Supporting Information 9-Azido Analogs of Three Sialic Acid Forms for Metabolic Remodeling of Cell-Surface Sialoglycans Bo Cheng,†,‡ Lu Dong,†,§ Yuntao Zhu,†,‡ Rongbing Huang,†,‡ Yuting Sun,†,‖ Qiancheng You,†,‡ Qitao Song,†,§ James C. Paton, ∇ Adrienne W. Paton,∇ and Xing Chen*,†,‡,§,⊥,# †College of Chemistry and Molecular Engineering, ‡Beijing National Laboratory for Molecular Sciences, §Peking−Tsinghua Center for Life Sciences,‖Academy for Advanced Interdisciplinary Studies, ⊥Synthetic and Functional Biomolecules Center, and #Key Laboratory of Bioorganic Chemistry and Molecular Engineering of Ministry of Education, Peking University, Beijing 100871, China ∇Research Centre for Infectious Diseases, Department of Molecular and Biomedical Science, University of Adelaide, Adelaide SA 5005, Australia Page S1 Table of Contents: Scheme S1.……………………………………………………….........……………. S3 Figure S1……………………………………………………..………..……………. S3 Figure S2……………………………………………………..………..…………… S4 Figure S3……………………………………………………..………..…………… S4 Figure S4……………………………………………………..………..…………… S5 Figure S5……………………………………………………..………..…………… S6 Figure S6……………………………………………………..………..…………….S7 Figure S7……………………………………………………..………..…………….S8 Figure S8……………………………………………………..………..…………….S9 Experimental Procedures……………………………….…........…………....S10-S27 Table S1………………………………………………..………..…………….S28-S48 Supporting Reference……………………………………………….......………...S49 Page S2 Scheme S1. Synthesis of 9AzNeu5Gc Figure S1: a, b, c, d) Representative scatter plots (FSC vs. SSC) and histograms of flow cytometry analysis -
Synopsis of the Heteroptera Or True Bugs of the Galapagos Islands
Synopsis of the Heteroptera or True Bugs of the Galapagos Islands ' 4k. RICHARD C. JROESCHNE,RD SMITHSONIAN CONTRIBUTIONS TO ZOOLOGY • NUMBER 407 SERIES PUBLICATIONS OF THE SMITHSONIAN INSTITUTION Emphasis upon publication as a means of "diffusing knowledge" was expressed by the first Secretary of the Smithsonian. In his formal plan for the Institution, Joseph Henry outlined a program that included the following statement: "It is proposed to publish a series of reports, giving an account of the new discoveries in science, and of the changes made from year to year in all branches of knowledge." This theme of basic research has been adhered to through the years by thousands of titles issued in series publications under the Smithsonian imprint, commencing with Smithsonian Contributions to Knowledge in 1848 and continuing with the following active series: Smithsonian Contributions to Anthropology Smithsonian Contributions to Astrophysics Smithsonian Contributions to Botany Smithsonian Contributions to the Earth Sciences Smithsonian Contributions to the Marine Sciences Smithsonian Contributions to Paleobiology Smithsonian Contributions to Zoology Smithsonian Folklife Studies Smithsonian Studies in Air and Space Smithsonian Studies in History and Technology In these series, the Institution publishes small papers and full-scale monographs that report the research and collections of its various museums and bureaux or of professional colleagues in the world of science and scholarship. The publications are distributed by mailing lists to libraries, universities, and similar institutions throughout the world. Papers or monographs submitted for series publication are received by the Smithsonian Institution Press, subject to its own review for format and style, only through departments of the various Smithsonian museums or bureaux, where the manuscripts are given substantive review. -
Dataset for the Quantitative Proteomics Analysis of the Primary Hepatocellular Carcinoma with Single and Multiple Lesions
Data in Brief 5 (2015) 226–240 Contents lists available at ScienceDirect Data in Brief journal homepage: www.elsevier.com/locate/dib Data Article Dataset for the quantitative proteomics analysis of the primary hepatocellular carcinoma with single and multiple lesions Xiaohua Xing a,b, Yao Huang c, Sen Wang a,b, Minhui Chi a,b,c, Yongyi Zeng a,b,c, Lihong Chen a,b,c, Ling Li a,b,c, Jinhua Zeng a,b,c, Minjie Lin a,b, Xiao Han d, Jingfeng Liu a,b,c, Xiaolong Liu a,b,n a The Liver Center of Fujian Province, Fujian Medical University, Fuzhou 350025, People’s Republic of China b The United Innovation of Mengchao Hepatobiliary Technology Key Laboratory of Fujian Province, Mengchao Hepatobiliary Hospital of Fujian Medical University, Fuzhou 350025, People’s Republic of China c Liver Disease Center, The First Affiliated Hospital of Fujian Medical University, Fuzhou 350007, People’s Republic of China d Biotechnology Research Institute, Chinese Academy of Agricultural Sciences, Beijing 100081, People’s Republic of China article info abstract Article history: Hepatocellular Carcinoma (HCC) is one of the most common malig- Received 18 August 2015 nant tumor, which is causing the second leading cancer-related death Received in revised form worldwide. The tumor tissues and the adjacent noncancerous tissues 27 August 2015 obtained from HCC patients with single and multiple lesions were Accepted 28 August 2015 quantified using iTRAQ. A total of 5513 proteins (FDR of 1%) were Available online 8 September 2015 identified which correspond to roughly 27% of the total liver proteome. -
Transcriptome Profiling Revealed Potentially
Wang et al. BMC Genomics (2020) 21:546 https://doi.org/10.1186/s12864-020-06950-y RESEARCH ARTICLE Open Access Transcriptome profiling revealed potentially important roles of defensive gene expression in the divergence of insect biotypes: a case study with the cereal aphid Sitobion avenae Da Wang1,2, Deguang Liu1,2* , Xiaoqin Shi1,2, Yujing Yang1,2, Na Zhang1,2 and Zheming Shang1,2 Abstract Background: Many insects can develop differential biotypes on variable host plants, but the underlying molecular factors and mechanisms are not well understood. To address this issue, transcriptome profiling analyses were conducted for two biotypes of the cereal aphid, Sitobion avenae (Fabricius), on both original and alternative plants. Results: Comparisons between both biotypes generated 4174 differentially expressed unigenes (DEGs). In their response to host plant shift, 39 DEGs were shared by both biotypes, whereas 126 and 861 DEGs occurred only in biotypes 1 and 3, respectively. MMC (modulated modularity clustering) analyses showed that specific DEGs of biotypes 1 and 3 clustered into five and nine transcriptional modules, respectively. Among these DEGs, defense- related genes underwent intensive expression restructuring in both biotypes. However, biotype 3 was found to have relatively lower gene transcriptional plasticity than biotype 1. Gene enrichment analyses of the abovementioned modules showed functional divergence in defensive DEGs for the two biotypes in response to host transfer. The expression plasticity for some defense related genes was showed to be directly related to fecundity of S. avenae biotypes on both original and alternative plants, suggesting that expression plasticity of key defensive genes could have significant impacts on the adaptive potential and differentiation of S. -
The Effect of Acid on the Dynamics of Intracellular Zinc and the Marker Expressions Of
The Effect of Acid on the Dynamics of Intracellular Zinc and the Marker Expressions of Pluripotency in Somatic Cells A thesis presented to the faculty of the College of Arts and Sciences of Ohio University In partial fulfillment of the requirements for the degree Master of Science Yuli Hu April 2021 © 2021 Yuli Hu. All Rights Reserved. 2 This thesis titled The Effect of Acid on the Dynamics of Intracellular Zinc and the Marker Expressions of Pluripotency in Somatic Cells by YULI HU has been approved for the Department of Biological Sciences and the College of Arts and Sciences by Yang V. Li Professor of Biomedical Sciences Florenz Plassmann Dean, College of Arts and Sciences 3 Abstract YULI HU, M.S., April 2021, Biological Sciences The Effect of Acid on the Dynamics of Intracellular Zinc and the Marker Expressions of Pluripotency in Somatic Cells Director of Thesis: Yang V. Li Microenvironmental pH is one of the factors that affect the stability of zinc- protein binding. The tight binding between zinc and proteins is favored by the basic pH, whereas acidic pH favors a loose bound, and treatment of strong acid results in the dissociation of zinc. Physiologically, the stomach uses a very acidic pH to digest food which results in a high amount of soluble zinc in the stomach. Whether or not zinc co- present with acid and the effect of zinc on the gastric lining has rarely been discussed. In my experiments, acidic treatment induced the expression of a pluripotent marker in primary cultured gastric cells. It also stimulated the release of intracellular zinc, suggesting that acidic pH supported protein expression through dynamic zinc regulation. -
Effects of a Bacterial ACC Deaminase on Plant Growth
Effects of a bacterial ACC deaminase on plant growth-promotion by Jennifer Claire Czarny A thesis presented to the University of Waterloo in fulfilment of the thesis requirement for the degree of Doctor of Philosophy in Biology Waterloo Ontario, Canada, 2008 c Jennifer Claire Czarny 2008 Author's declaration I hereby declare that I am the sole author of this thesis. This is a true copy of the thesis, including any required final revisions, as accepted by my examiners. I understand that my thesis may be made electronically available to the public. ii Abstract Plants often live in association with growth-promoting bacteria, which provide them with several benefits. One such benefit is the lowering of plant ethylene levels through the action of the bacterial enzyme 1-aminocyclopropane-1-carboxylic acid (ACC) deaminase that cleaves the immediate biosynthetic precursor of ethylene, ACC. The plant hormone ethylene is responsible for many aspects of plant growth and development but under stressful conditions ethylene exacerbates stress symptoms. The ACC deaminase-containing bacterium Pseudomonas putida UW4, isolated from the rhizosphere of reeds, is a potent plant growth- promoting strain and as such was used, along with an ACC deaminase minus mutant of this strain, to study the role of ACC deaminase in plant growth-promotion. Also, transgenic plants expressing a bacterial ACC deaminase gene were used to study the role of this enzyme in plant growth and stress tolerance in the presence and absence of nickel. Transcriptional changes occurring within plant tissues were investigated with the use of an Arabidopsis oligonucleotide microarray. The results showed that transcription of genes involved in hormone regulation, secondary metabolism and the stress response changed in all treatments. -
Synopsis and Keys to the Tribes, Genera, and Species of Miridae (Hemiptera: Heteroptera) of Minas Gerais, Brazil Part I: Bryocorinae
Zootaxa 2920: 1–41 (2011) ISSN 1175-5326 (print edition) www.mapress.com/zootaxa/ Article ZOOTAXA Copyright © 2011 · Magnolia Press ISSN 1175-5334 (online edition) Synopsis and keys to the tribes, genera, and species of Miridae (Hemiptera: Heteroptera) of Minas Gerais, Brazil Part I: Bryocorinae PAULO SERGIO FIUZA FERREIRA1 & THOMAS J. HENRY2 1Museu de Entomologia, Departamento de Biologia Animal, Universidade Federal de Viçosa, 36570-000, Viçosa, Brazil. E-mail: [email protected] 2Systematic Entomology Laboratory, Agricultural Research Service, United States Department of Agriculture, c/o National Museum of Natural History, Smithsonian Institution, Washington, D.C., USA. E-mail: [email protected] Table of contents Abstract . 3 Introduction . 3 Material and methods . 4 Minas Gerais geography . 4 Taxonomic synopsis . 5 Subfamily Bryocorinae Baerensprung . 5 Key to Minas Gerais Tribes of Bryocorinae . 5 Tribe Bryocorini Baerensprung . 6 Genus Monalocoris Dahlbom . 6 Key to species of Monalocoris of Minas Gerais . 6 Monalocoris carioca Carvalho and Gomes . 6 Monalocoris pallidiceps (Reuter) . 6 Tribe Dicyphini Reuter . 7 Key to the genera of Dicyphini of Minas Gerais . 7 Genus Campyloneuropsis Poppius . 7 Key to Minas Gerais species of Campyloneuropsis . 7 Campyloneuropsis infumatus (Carvalho) . 7 Campyloneuropsis nigroculatus (Carvalho) . 8 Genus Engytatus Reuter . 8 Key to the Minas Gerais species of Engytatus . 8 Engytatus modestus (Distant) . 8 Engytatus varians (Distant) . 9 Genus Macrolophus Fieber . 9 Key to Minas Gerais species of Macrolophus . 9 Macrolophus aragarsanus Carvalho . 10 Macrolophus basicornis (Stål) . 10 Macrolophus cuibanus Carvalho . 10 Macrolophus praeclarus (Distant) . 11 Genus Tupiocoris China and Carvalho . 11 Key to the Minas Gerais species of Tupiocoris. 11 Tupiocoris cucurbitaceus (Spinola) . -
Surveying for Terrestrial Arthropods (Insects and Relatives) Occurring Within the Kahului Airport Environs, Maui, Hawai‘I: Synthesis Report
Surveying for Terrestrial Arthropods (Insects and Relatives) Occurring within the Kahului Airport Environs, Maui, Hawai‘i: Synthesis Report Prepared by Francis G. Howarth, David J. Preston, and Richard Pyle Honolulu, Hawaii January 2012 Surveying for Terrestrial Arthropods (Insects and Relatives) Occurring within the Kahului Airport Environs, Maui, Hawai‘i: Synthesis Report Francis G. Howarth, David J. Preston, and Richard Pyle Hawaii Biological Survey Bishop Museum Honolulu, Hawai‘i 96817 USA Prepared for EKNA Services Inc. 615 Pi‘ikoi Street, Suite 300 Honolulu, Hawai‘i 96814 and State of Hawaii, Department of Transportation, Airports Division Bishop Museum Technical Report 58 Honolulu, Hawaii January 2012 Bishop Museum Press 1525 Bernice Street Honolulu, Hawai‘i Copyright 2012 Bishop Museum All Rights Reserved Printed in the United States of America ISSN 1085-455X Contribution No. 2012 001 to the Hawaii Biological Survey COVER Adult male Hawaiian long-horned wood-borer, Plagithmysus kahului, on its host plant Chenopodium oahuense. This species is endemic to lowland Maui and was discovered during the arthropod surveys. Photograph by Forest and Kim Starr, Makawao, Maui. Used with permission. Hawaii Biological Report on Monitoring Arthropods within Kahului Airport Environs, Synthesis TABLE OF CONTENTS Table of Contents …………….......................................................……………...........……………..…..….i. Executive Summary …….....................................................…………………...........……………..…..….1 Introduction ..................................................................………………………...........……………..…..….4 -
Frontiersin.Org 1 April 2015 | Volume 9 | Article 123 Saunders Et Al
ORIGINAL RESEARCH published: 28 April 2015 doi: 10.3389/fnins.2015.00123 Influx mechanisms in the embryonic and adult rat choroid plexus: a transcriptome study Norman R. Saunders 1*, Katarzyna M. Dziegielewska 1, Kjeld Møllgård 2, Mark D. Habgood 1, Matthew J. Wakefield 3, Helen Lindsay 4, Nathalie Stratzielle 5, Jean-Francois Ghersi-Egea 5 and Shane A. Liddelow 1, 6 1 Department of Pharmacology and Therapeutics, University of Melbourne, Parkville, VIC, Australia, 2 Department of Cellular and Molecular Medicine, University of Copenhagen, Copenhagen, Denmark, 3 Walter and Eliza Hall Institute of Medical Research, Parkville, VIC, Australia, 4 Institute of Molecular Life Sciences, University of Zurich, Zurich, Switzerland, 5 Lyon Neuroscience Research Center, INSERM U1028, Centre National de la Recherche Scientifique UMR5292, Université Lyon 1, Lyon, France, 6 Department of Neurobiology, Stanford University, Stanford, CA, USA The transcriptome of embryonic and adult rat lateral ventricular choroid plexus, using a combination of RNA-Sequencing and microarray data, was analyzed by functional groups of influx transporters, particularly solute carrier (SLC) transporters. RNA-Seq Edited by: Joana A. Palha, was performed at embryonic day (E) 15 and adult with additional data obtained at University of Minho, Portugal intermediate ages from microarray analysis. The largest represented functional group Reviewed by: in the embryo was amino acid transporters (twelve) with expression levels 2–98 times Fernanda Marques, University of Minho, Portugal greater than in the adult. In contrast, in the adult only six amino acid transporters Hanspeter Herzel, were up-regulated compared to the embryo and at more modest enrichment levels Humboldt University, Germany (<5-fold enrichment above E15).