Accurate Rest-Frequencies of Ketenimine (CH2CNH) at Submillimetre Wavelength
Total Page:16
File Type:pdf, Size:1020Kb
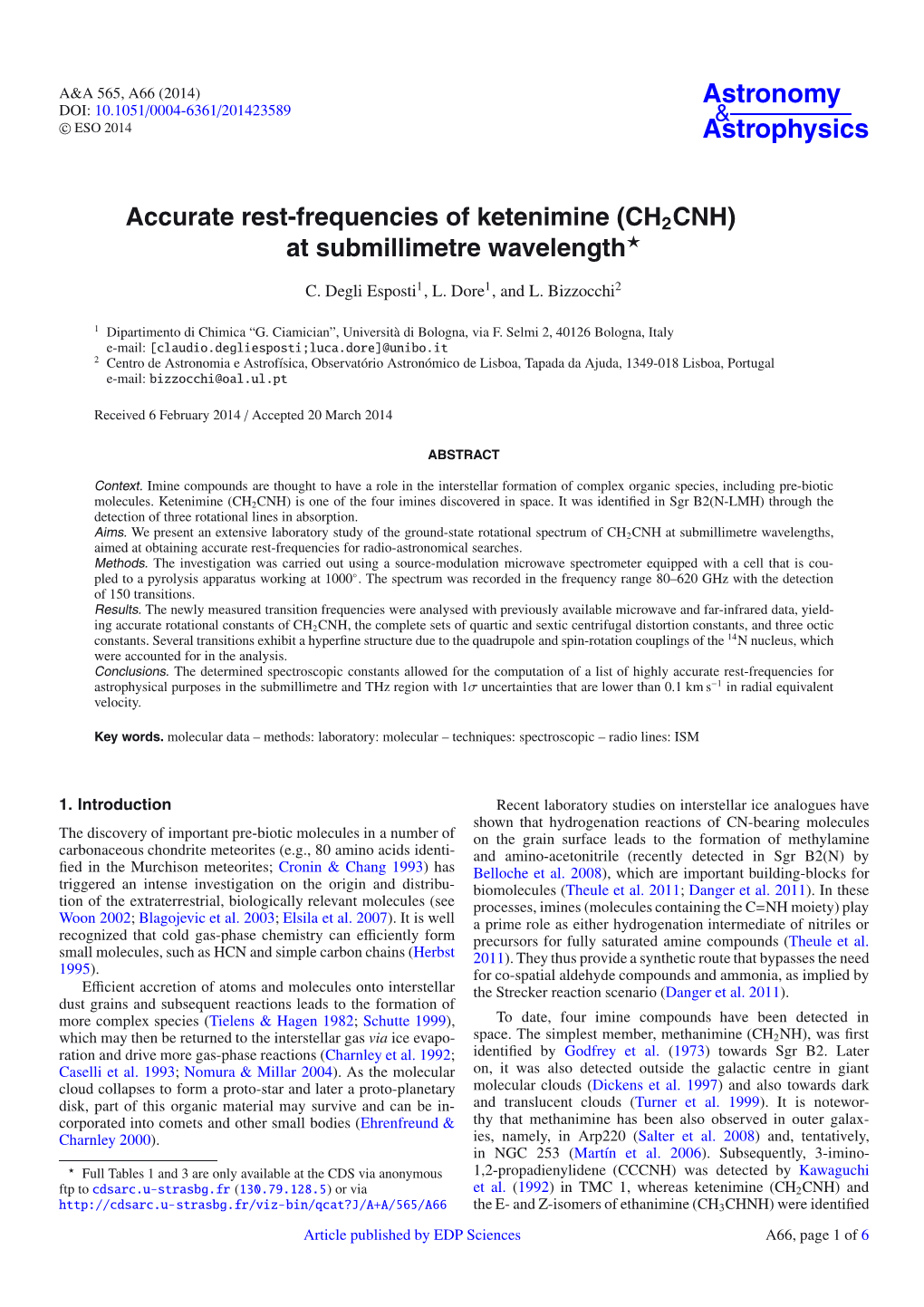
Load more
Recommended publications
-
Accurate Enthalpies of Formation of Astromolecules: Energy, Stability and Abundance
Accurate Enthalpies of Formation of Astromolecules: Energy, Stability and Abundance Emmanuel E. Etim and Elangannan Arunan* Inorganic and Physical Chemistry Department, Indian Institute of Science Bangalore, India-560012 *email: [email protected] ABSTRACT: Accurate enthalpies of formation are reported for known and potential astromolecules using high level ab initio quantum chemical calculations. A total of 130 molecules comprising of 31 isomeric groups and 24 cyanide/isocyanide pairs with atoms ranging from 3 to 12 have been considered. The results show an interesting, surprisingly not well explored, relationship between energy, stability and abundance (ESA) existing among these molecules. Among the isomeric species, isomers with lower enthalpies of formation are more easily observed in the interstellar medium compared to their counterparts with higher enthalpies of formation. Available data in literature confirm the high abundance of the most stable isomer over other isomers in the different groups considered. Potential for interstellar hydrogen bonding accounts for the few exceptions observed. Thus, in general, it suffices to say that the interstellar abundances of related species are directly proportional to their stabilities. The immediate consequences of this relationship in addressing some of the whys and wherefores among astromolecules and in predicting some possible candidates for future astronomical observations are discussed. Our comprehensive results on 130 molecules indicate that the available experimental enthalpy -
Possible Gas-Phase Syntheses for Seven Neutral Molecules Studied Recently with the Green Bank Telescope
A&A 474, 521–527 (2007) Astronomy DOI: 10.1051/0004-6361:20078246 & c ESO 2007 Astrophysics Possible gas-phase syntheses for seven neutral molecules studied recently with the Green Bank Telescope D. Quan1 and E. Herbst2 1 Chemical Physics Program, The Ohio State University, Columbus, Ohio 43210, USA e-mail: [email protected] 2 Departments of Physics, Chemistry and Astronomy, The Ohio State University, Columbus, OH 43210, USA Received 9 July 2007 / Accepted 17 August 2007 ABSTRACT Aims. With the Green Bank telescope (GBT), seven neutral molecules have been newly detected or confirmed towards either the cold interstellar core TMC-1 or the hot core source Sgr B2(N) within the last 1–2 years. Towards TMC-1, the new molecules seen are cyanoallene (CH2CCHCN) and methyl triacetylene (CH3C6H) while methyl cyanoacetylene (CH3CCCN) and methyl cyanodiacety- lene (CH3C5N) were confirmed. Towards Sgr B2(N), the three newly detected molecules are cyclopropenone (c-C3H2O), ketenimine (CH2CNH), and acetamide (CH3CONH2); these are mainly seen in absorption and are primarily located in an envelope around the hot core. In this work, we report a detailed study of the gas-phase chemistry of all seven molecules. Methods. Starting with our updated gas-phase chemical reaction network osu.01.2007, we added formation and depletion reactions to treat the chemistry of each of the seven molecules. Some of these were already in our network but with incomplete chemistry, while most were not in the network at all prior to this work. We assumed the standard physical conditions for TMC-1 and assumed that these also hold for the envelope around Sgr B2(N). -
Hydroxyacetonitrile (HOCH2CN) As a Precursor for Formylcyanide (CHOCN), Ketenimine (CH2CNH), and Cyanogen (NCCN) in Astrophysical Conditions
A&A 549, A93 (2013) Astronomy DOI: 10.1051/0004-6361/201219779 & c ESO 2013 Astrophysics Hydroxyacetonitrile (HOCH2CN) as a precursor for formylcyanide (CHOCN), ketenimine (CH2CNH), and cyanogen (NCCN) in astrophysical conditions G. Danger1, F. Duvernay1, P. Theulé1, F. Borget1, J.-C. Guillemin2, and T. Chiavassa1 1 Aix-Marseille Univ, CNRS, PIIM UMR 7345, 13397 Marseille, France e-mail: [email protected] 2 Institut des Sciences Chimiques de Rennes, École Nationale Supérieure de Chimie de Rennes, CNRS, UMR 6226, Avenue du Général Leclerc, CS 50837, 35708 Rennes Cedex 7, France Received 8 June 2012 / Accepted 19 November 2012 ABSTRACT Context. The reactivity in astrophysical environments can be investigated in the laboratory through experimental simulations, which provide understanding of the formation of specific molecules detected in the solid phase or in the gas phase of these environments. In this context, the most complex molecules are generally suggested to form at the surface of interstellar grains and to be released into the gas phase through thermal or non-thermal desorption, where they can be detected through rotational spectroscopy. Here, we focus our experiments on the photochemistry of hydroxyacetonitrile (HOCH2CN), whose formation has been shown to compete with aminomethanol (NH2CH2OH), a glycine precursor, through the Strecker synthesis. Aims. We present the first experimental investigation of the ultraviolet (UV) photochemistry of hydroxyacetonitrile (HOCH2CN) as a pure solid or diluted in water ice. Methods. We used Fourier transform infrared (FT-IR) spectroscopy to characterize photoproducts of hydroxyacetonitrile (HOCH2CN) and to determine the different photodegradation pathways of this compound. To improve the photoproduct identifications, irradiations of hydroxyacetonitrile 14N and 15N isotopologues were performed, coupled with theoretical calculations. -
The Prebiotic Molecular Inventory of Serpens SMM1
Astronomy & Astrophysics manuscript no. SMM1_C2H3NO_isomers ©ESO 2021 January 1, 2021 The prebiotic molecular inventory of Serpens SMM1 I. An investigation of the isomers CH3NCO and HOCH2CN N.F.W. Ligterink1, A. Ahmadi2, A. Coutens3, Ł. Tychoniec2 H. Calcutt4; 5, E.F. van Dishoeck2; 6, H. Linnartz7, J.K. Jørgensen8, R.T. Garrod9, and J. Bouwman7 1 Physics Institute, University of Bern, Sidlerstrasse 5, 3012 Bern, Switzerland e-mail: [email protected] 2 Leiden Observatory, Leiden University, PO Box 9513, 2300 RA Leiden, The Netherlands 3 Laboratoire d’Astrophysique de Bordeaux, Univ. Bordeaux, CNRS, B18N, allée Geoffroy Saint-Hilaire, 33615 Pessac, France 4 Department of Space, Earth and Environment, Chalmers University of Technology, 41296, Gothenburg, Sweden 5 Institute of Astronomy, Faculty of Physics, Astronomy and Informatics, Nicolaus Copernicus University, Grudziadzka 5, 87-100 Torun, Poland 6 Max-Planck Institut für Extraterrestrische Physik (MPE), Giessenbachstr. 1, 85748 Garching, Germany 7 Laboratory for Astrophysics, Leiden Observatory, Leiden University, PO Box 9513, 2300 RA Leiden, The Netherlands 8 Centre for Star and Planet Formation, Niels Bohr Institute & Natural History Museum of Denmark, University of Copenhagen, Øster Voldgade 5–7, 1350 Copenhagen K., Denmark 9 Departments of Chemistry and Astronomy, University of Virginia, Charlottesville, VA 22904, USA Received October 8, 2020; accepted December 22, 2020 ABSTRACT Aims. Methyl isocyanate (CH3NCO) and glycolonitrile (HOCH2CN) are isomers and prebiotic molecules that are involved in the formation of peptide structures and the nucleobase adenine, respectively. These two species are investigated to study the interstellar chemistry of cyanides (CN) and isocyanates (NCO) and to gain insight into the reservoir of interstellar prebiotic molecules. -
Dimethyl Sulfoxide Oxidation of Primary Alcohols
Western Michigan University ScholarWorks at WMU Master's Theses Graduate College 8-1966 Dimethyl Sulfoxide Oxidation of Primary Alcohols Carmen Vargas Zenarosa Follow this and additional works at: https://scholarworks.wmich.edu/masters_theses Part of the Chemistry Commons Recommended Citation Zenarosa, Carmen Vargas, "Dimethyl Sulfoxide Oxidation of Primary Alcohols" (1966). Master's Theses. 4374. https://scholarworks.wmich.edu/masters_theses/4374 This Masters Thesis-Open Access is brought to you for free and open access by the Graduate College at ScholarWorks at WMU. It has been accepted for inclusion in Master's Theses by an authorized administrator of ScholarWorks at WMU. For more information, please contact [email protected]. DIMETHYL SULFOXIDE OXIDATION OF PRIMARY ALCOHOLS by Carmen Vargas Zenarosa A thesis presented to the Faculty of the School of Graduate Studies in partial fulfillment of the Degree of Master of Arts Western Michigan University Kalamazoo, Michigan August, 1966 ACKNOWLEDGMENTS The author wishes to express her appreciation to the members of her committee, Dr, Don C. Iffland and Dr. Donald C, Berndt, for their helpful suggestions and most especially to Dr, Robert E, Harmon for his patience, understanding, and generous amount of time given to insure the completion of this work. Appreciation is also expressed for the assistance given by her. colleagues. The author acknowledges the assistance given by the National Institutes 0f Health for this research project. Carmen Vargas Zenarosa ii TABLE OF CONTENTS Page -
Ketenes 25/01/2014 Part 1
Baran Group Meeting Hai Dao Ketenes 25/01/2014 Part 1. Introduction Ph Ph n H Pr3N C A brief history Cl C Ph + nPr NHCl Ph O 3 1828: Synthesis of urea = the starting point of modern organic chemistry. O 1901: Wedekind's proposal for the formation of ketene equivalent (confirmed by Staudinger 1911) Wedekind's proposal (1901) 1902: Wolff rearrangement, Wolff, L. Liebigs Ann. Chem. 1902, 325, 129. 2 Wolff adopt a ketene structure in 1912. R 2 hν R R2 1905: First synthesis and characterization of a ketene: in an efford to synthesize radical 2, 1 ROH R C Staudinger has synthesized diphenylketene 3, Staudinger, H. et al., Chem. Ber. 1905, 1735. N2 1 RO CH or Δ C R C R1 1907-8: synthesis and dicussion about structure of the parent ketene, Wilsmore, O O J. Am. Chem. Soc. 1907, 1938; Wilsmore and Stewart Chem. Ber. 1908, 1025; Staudinger and Wolff rearrangement (1902) O Klever Chem. Ber. 1908, 1516. Ph Ph Cl Zn Ph O hot Pt wire Zn Br Cl Cl CH CH2 Ph C C vs. C Br C Ph Ph HO O O O O O O O 1 3 (isolated) 2 Wilsmore's synthesis and proposal (1907-8) Staudinger's synthesis and proposal (1908) wanted to make Staudinger's discovery (1905) Latest books: ketene (Tidwell, 1995), ketene II (Tidwell, 2006), Science of Synthesis, Vol. 23 (2006); Latest review: new direactions in ketene chemistry: the land of opportunity (Tidwell et al., Eur. J. Org. Chem. 2012, 1081). Search for ketenes, Google gave 406,000 (vs. -
Effects of Metal Ions in Free Radical Reactions Richard Duane Kriens Iowa State University
Iowa State University Capstones, Theses and Retrospective Theses and Dissertations Dissertations 1963 Effects of metal ions in free radical reactions Richard Duane Kriens Iowa State University Follow this and additional works at: https://lib.dr.iastate.edu/rtd Part of the Organic Chemistry Commons Recommended Citation Kriens, Richard Duane, "Effects of metal ions in free radical reactions " (1963). Retrospective Theses and Dissertations. 2544. https://lib.dr.iastate.edu/rtd/2544 This Dissertation is brought to you for free and open access by the Iowa State University Capstones, Theses and Dissertations at Iowa State University Digital Repository. It has been accepted for inclusion in Retrospective Theses and Dissertations by an authorized administrator of Iowa State University Digital Repository. For more information, please contact [email protected]. This dissertation has been 64—3880 microfilmed exactly as received KRIENS, Richard Duane, 1932- EFFECTS OF METAL IONS IN FREE RADICAL REACTIONS. Iowa State University of Science and Technology Ph.D„ 1963 Chemistry, organic University Microfilms, Inc., Ann Arbor, Michigan EFFECTS OF METAL IONS IN FREE RADICAL REACTIONS by Richard Duane Kriens A Dissertation Submitted to the Graduate Faculty in Partial Fulfillment of The Requirements for the Degree of DOCTOR OF PHILOSOPHY Major Subject: Organic Chemistry Approved: Signature was redacted for privacy. In Charge of Major Work Signature was redacted for privacy. ead of Major Departmei^ Signature was redacted for privacy. Iowa State University Of Science and Technology Ames, Iowa 1963 11 TABLE OF CONTENTS Page PART I. REACTIONS OF RADICALS WITH METAL SALTS. ... 1 INTRODUCTION 2 REVIEW OF LITERATURE 3 RESULTS AND DISCUSSION 17 EXPERIMENTAL 54 Chemicals 54 Apparatus and Procedure 66 Reactions of compounds with the 2-cyano-2-propyl radical 66 Reactions of compounds with the phenyl radical 67 Procedure for Sandmeyer type reaction. -
Chemodivergent Conversion of Ketenimines Bearing Cyclic
Page 1 of 18 The Journal of Organic Chemistry This document is the Accepted Manuscript version of a Published Work that appeared in final form in the Journal Of Organic Chemistry, copyright © American Chemical Society, after peer review and technical editing by the publisher. To access the final edited and published work see https://pubs.acs.org/doi/full/10.1021/acs.joc.9b01014 1 2 Chemodivergent Conversion of Ketenimines Bearing Cyclic Dithioacetalic Units into 3 Isoquinoline-1-thiones or Quinolin-4-ones as a Function of the Acetalic Ring-Size 4 5 Mateo Alajarin†, Marta Marin-Luna,‡ Pilar Sanchez-Andrada*,†, and Angel Vidal†¥ 6 7 8 † Department of Organic Chemistry, Faculty of Chemistry. University of Murcia. 9 Regional Campus of International Excellence "Campus Mare Nostrum", 30100 Murcia, Spain 10 11 E-mail: [email protected] 12 13 ‡Departamento de Química Orgánica, Universidade de Vigo, Campus Lagoas-Marcosende, 36310 Vigo, Spain 14 ¥ 15 Recently passed away 16 17 18 ORCID ID’s: Prof. M. Alajarin 000-0002-7112-5578, Dr. M. Marin-Luna 0000-0003-3531-6622 Prof. P. Sanchez Andrada 000-0002-9944-8563, 19 Prof. A. Vidal 000-0002-4347-6215. 20 21 22 23 24 25 Abstract 26 27 C-Alkoxycarbonyl-C-phenyl-N-aryl ketenimines bearing 1,3-dithiolan-2-yl or 1,3-dithian-2-yl substituents at ortho-position of the C-phenyl ring 28 respectively transform into isoquinoline-1-thiones and quinolin-4-ones under thermal treatment in toluene solution. The formation of 29 isoquinolinethiones involves a rare degradation of the 1,3-dithiolane ring whereas, in contrast, the 1,3-dithiane ring remains intact during the 30 reaction course leading to quinolin-4-ones. -
Chemical Modeling of Interstellar Molecules in Dense Cores
Chemical Modeling of Interstellar Molecules in Dense Cores Dissertation Presented in Partial Fulfillment of the Requirements for the Degree Doctor of Philosophy in the Graduate School of The Ohio State University By Donghui Quan, M.S. Graduate Program in Chemical Physics The Ohio State University 2009 Dissertation Committee: Professor Eric Herbst, Advisor Professor Frank C. De Lucia Professor Anil K. Pradhan Copyright by Donghui Quan 2009 ii Abstract There are billions of stars in our galaxy, the Milky Way Galaxy. In between the stars is where the so-called “interstellar medium” locates. The majority of the mass of interstellar medium is clumped into interstellar clouds, in which cold and hot dense cores exist. Despite of the extremely low densities and low temperatures of the dense cores, over one hundred molecules have been found in these sources. This makes the field of astrochemistry vivid. Chemical modeling plays very important roles to understand the mechanism of formation and destruction of interstellar molecules. In this thesis, chemical kinetics models of different types were applied: in Chapter 4, pure gas phase models were used for seven newly detected or confirmed molecules by the Green Bank Telescope; in Chapter 5, the potential reason of non-detection of O2 was explored; in Chapter 6, the mysterious behavior of CHNO and CHNS isomers were studied by gas-grain models. In addition, effects of varying rate coefficients to the models are also discussed in Chapter 3 and 7. ii Dedication Dedicated to my parents Quan He (全和), Li Lianxiang (李廉祥) and my wife Wang Jing (王璟) iii Acknowledgements First of all, I would like to thank my advisor, Professor Eric Herbst, who has always been supportive in all means: a mentor in my graduate study, a guide in astrochemical research, and a kind “father” in the life. -
A Three-Component Synthesis of Functionalized Ketenimines by The
J. Serb. Chem. Soc. 76 (1) 13–20 (2011) UDC 547.48+547.467+547.313+ JSCS–4094 547.239+547.421:542.9 Original scientific paper A three-component synthesis of functionalized ketenimines by the reaction of alkyl isocyanides and dialkyl acetylene- dicarboxylates in the presence of 2-quinolinol BITA MOHTAT1*, HOORIEH DJAHANIANI2, ISSA YAVARI3 and KOBRA NADERI1 1Chemistry Department, Islamic Azad University, Karaj Branch, Karaj, 2Chemistry Department, Islamic Azad University, East Branch, Qiamdasht, Tehran and 3Chemistry Department, Islamic Azad University, Science and Research Branch, Tehran, Iran (Received 18 February, revised 21 June 2010) Abstract: The 1:1 reactive intermediates generated by the addition of alkyl isocyanides to dialkyl acetylenedicarboxylates were trapped by 2-quinolinol to yield highly functionalized ketenimines and, in some cases, minor amounts of 1-azabuta-1,3-dienes. Keywords: ketenimines; 1-azadienes; alkyl isocyanides; acetylenic esters; NH- -acids; multi-component reaction. INTRODUCTION Ketenimines are important reactive intermediates that occur as transient compounds in many thermal and photochemical reactions.1 There has been in- tense interest in their reactions, such as cycloaddition,2 nucleophilic3,4 and elec- trophilic addition.5 They have also found widespread use as reactive starting materials for the formation of four-, five-, and six-membered heterocyclic ring systems.5–7 Methods for the synthesis of ketenimines have been extensively re- viewed.8 The addition of nucleophilic carbenes, such as isocyanides, to dialkyl acetylenedicarboxylates was investigated in detail.9 The trapping of the 1:1 in- termediate formed between dialkyl acetylenedicarboxylates and isocyanides with OH, NH, and CH acids has been widely studied.10–14 In continuation of our in- terest in the application of isocyanides in multi-component reactions, MCR,15–18 an efficient synthesis of ketenimine 4 from alkyl isocyanides 1 and dialkyl ace- tylenedicarboxylates 2 in the presence of a strong NH-acid, such as quinolin-2-ol, is reported herein. -
Photochemistry of 2-Formylphenylnitrene: a Doorway
Article Cite This: J. Am. Chem. Soc. XXXX, XXX, XXX-XXX pubs.acs.org/JACS Photochemistry of 2‑Formylphenylnitrene: A Doorway to Heavy- Atom Tunneling of a Benzazirine to a Cyclic Ketenimine † † ‡ § † Claudió M. Nunes,*, Igor Reva, Sebastian Kozuch, Robert J. McMahon, and Rui Fausto † CQC, Department of Chemistry, University of Coimbra, 3004-535 Coimbra, Portugal ‡ Department of Chemistry, Ben-Gurion University of the Negev, Beer-Sheva 841051, Israel § Department of Chemistry, University of Wisconsin-Madison, Madison, Wisconsin 53706-1322, United States *S Supporting Information ABSTRACT: The slippery potential energy surface of aryl nitrenes has revealed unexpected and fascinating reactions. To explore such a challenging surface, one powerful approach is to use a combination of a cryogenic matrix environment and a tunable narrowband radiation source. In this way, we discovered the heavy-atom tunneling reaction involving spontaneous ring expansion of a fused-ring benzazirine into a seven-membered ring cyclic ketenimine. The benzazirine was generated in situ by the photochemistry of protium and deuterated triplet 2‑formylphenylnitrene isolated in an argon matrix. The ring- expansion reaction takes place at 10 K with a rate constant of ∼7.4 × 10−7 s−1, despite an estimated activation barrier of 7.5 kcal mol−1. Moreover, it shows only a marginal increase in the rate upon increase of the absolute temperature by a factor of 2. Computed rate constants with and without tunneling confirm that the reaction can only occur by a tunneling process from the ground state at cryogenic conditions. It was also found that the ring- expansion reaction rate is more than 1 order of magnitude faster when the sample is exposed to broadband IR radiation. -
Deoxyribose-5-Phosphate Aldolase As a Synthetic Catalyst'
J. Am. Chem. SOC.1990, 112, 2013-2014 2013 chemical shift for the iminosilaacyl carbon at 6 299.07.3a3b Ad- Deoxyribose-5-phosphateAldolase as a Synthetic dition of 1 equiv of CN(XyI) to a benzene solution of 4, or reaction Catalyst' of 1 with 2 equiv of CN(Xyl), results in formation of a blue complex 5. The combustion analysis of isolated 5 is consistent Carlos F. Barbas, 111, Yi-Fong Wang, and Chi-Huey Wong* with a 1:2 adduct of 1 with isocyanide, Cp,Sc(CN(Xyl)),Si- (SiMe3)3. However, 'H NMR data for 5 indicate a complex Department of Chemistry structure and the presence of three inequivalent SiMe3 groups in The Research Institute of Scripps Clinic a 1 :1: 1 ratioss Formation of X-ray-quality, blue crystals from La Jolla, California 92037 diethvl ether allowed comdete characterization of this comwund. Received November 29, 1989 Tie crystal structure7'(Figure 1) shows that 5 is the pioduct of an isocyanide-coupling reaction that results in further rear- Enzyme-catalyzed stereocontrolled aldol condensations are rangements. The structure drawn in Scheme I reflects the ob- valuable in organic synthesis, particularly in the synthesis of served structural parameters. The chelate ring of 5 is derived from carbohydrates and related s~bstances?~~We report here an initial the two isoc anide groups and contains a Sc( 1)-N( 1) single bond study on the synthetic utility of a bacterial 2-deoxyribose-5- (2.133 (7) i),a longer (dative) Sc(l)-N(2) bond (2.324 (8) A), phosphate aldolase (DERA, EC 4.1.2.4) overexpressed in Es- and a C=C double bond (C(l)-C(2) = 1.375 (12) A).