Quantum Emitters in Low Dimensional Van Der Waals Systems
Total Page:16
File Type:pdf, Size:1020Kb
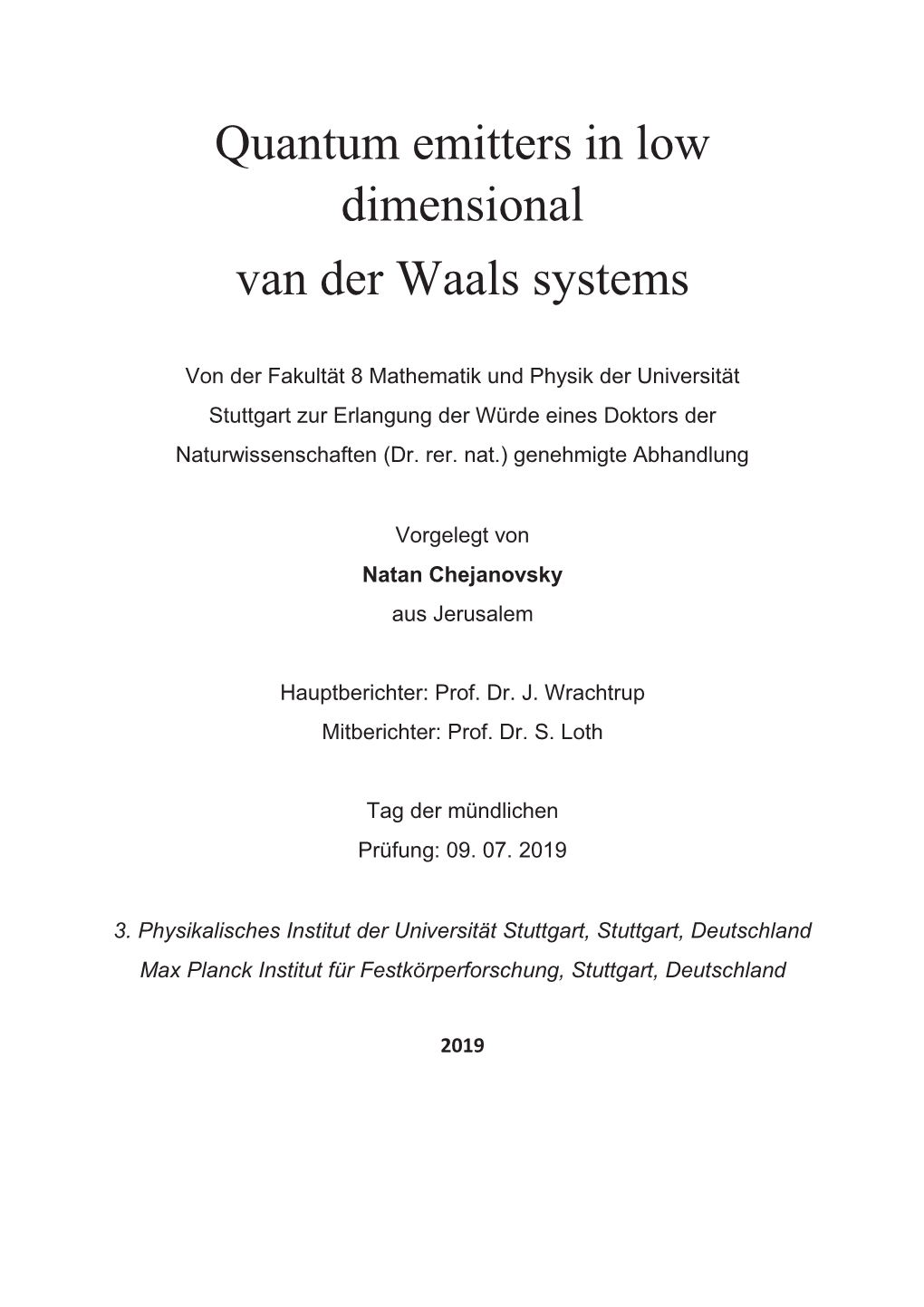
Load more
Recommended publications
-
Thesis Submitted for the Degree of Doctor of Philosophy
University of Bath PHD Development of Single-Source CVD Precursors for Group IV, V and VI Metal Disulfides Thompson, Joseph Award date: 2017 Awarding institution: University of Bath Link to publication Alternative formats If you require this document in an alternative format, please contact: [email protected] General rights Copyright and moral rights for the publications made accessible in the public portal are retained by the authors and/or other copyright owners and it is a condition of accessing publications that users recognise and abide by the legal requirements associated with these rights. • Users may download and print one copy of any publication from the public portal for the purpose of private study or research. • You may not further distribute the material or use it for any profit-making activity or commercial gain • You may freely distribute the URL identifying the publication in the public portal ? Take down policy If you believe that this document breaches copyright please contact us providing details, and we will remove access to the work immediately and investigate your claim. Download date: 11. Oct. 2021 Development of Single-Source CVD Precursors for Group IV, V and VI Metal Disulfides Joseph Robert Thompson A thesis submitted for the degree of Doctor of Philosophy University of Bath Department of Chemistry October 2016 COPYRIGHT Attention is drawn to the fact that copyright of this thesis/portfolio rests with the author and copyright of any previously published materials included may rest with third parties. A copy of this thesis/portfolio has been supplied on condition that anyone who consults it understands that they must not copy it or use material from it except as permitted by law or with the consent of the author or other copyright owners, as applicable. -
Download This Article PDF Format
Nanoscale View Article Online PAPER View Journal | View Issue Large-area tungsten disulfide for ultrafast photonics Cite this: Nanoscale, 2017, 9, 1871 Peiguang Yan,*a Hao Chen,a Jinde Yin,a Zihan Xu,b Jiarong Li,a Zike Jiang,a Wenfei Zhang,a Jinzhang Wang,a Irene Ling Li,a Zhipei Sunc and Shuangchen Ruan*a Two-dimensional (2D) layered transition metal dichalcogenides (TMDs) have attracted significant interest in various optoelectronic applications due to their excellent nonlinear optical properties. One of the most important applications of TMDs is to be employed as an extraordinary optical modulation material (e.g., the saturable absorber (SA)) in ultrafast photonics. The main challenge arises while embedding TMDs into fiber laser systems to generate ultrafast pulse trains and thus constraints their practical applications. Herein, few-layered WS2 with a large-area was directly transferred on the facet of the pigtail and acted as a SA for erbium-doped fiber laser (EDFL) systems. In our study, WS2 SA exhibited remarkable nonlinear optical properties (e.g., modulation depth of 15.1% and saturable intensity of 157.6 MW cm−2) and was Creative Commons Attribution 3.0 Unported Licence. used for ultrafast pulse generation. The soliton pulses with remarkable performances (e.g., ultrashort pulse duration of 1.49 ps, high stability of 71.8 dB, and large pulse average output power of 62.5 mW) Received 25th November 2016, could be obtained in a telecommunication band. To the best of our knowledge, the average output Accepted 29th December 2016 power of the mode-locked pulse trains is the highest by employing TMD materials in fiber laser systems. -
United States Patent (19) (11) 4,208,474 Jacobson Et Al
United States Patent (19) (11) 4,208,474 Jacobson et al. 45) Jun. 17, 1980 (54 CELL CONTAINING ALKALI METAL ANODE, CATHODE AND ALKALI OTHER PUBLICATIONS METAL-METALCHALCOGENEDE Hellstrom et al, Phase Relations and Charge Transport COMPOUND SOLD ELECTROLYTE in Ternary Aluminum Sulfides, Extended Abstracts, vol. 78-1, Electrochemical Soc., pp. 393-395, May 75) Inventors: Allan J. Jacobson, Princeton; 1978. Bernard G. Sibernagel, Scotch Plains, both of N.J. Primary Examiner-Donald L. Walton Attorney, Agent, or Firm-Kenneth P. Glynn 73) Assignee: Exxon Research & Engineering Co., Florham Park, N.J. 57 ABSTRACT A novel electrochemical cell is disclosed which con (21) Appl. No.: 974,021 tains an alkali metal anode, an electrolyte and a chalco genide cathode wherein the electrolyte is a solid com 22 Filed: Dec. 28, 1978 position containing an electrolytically active amount of 5ll int. Cl’............................................. HOM 6/18 one or more compounds having the formula: 52 U.S. C. ..................................... 429/191; 429/218 58 Field of Search ................................ 429/19, 218 56) References Cited wherein A is an alkali metal, wherein B is an element U.S. PATENT DOCUMENTS selected from the group consisting of boron and alumi 3,751,298 8/1973 Senderoff............................. 136/6 F num, wherein C is a chalcogen, wherein x and y are 3,791,867 2/1974 Broadhead et al. ................. 36/6 F each greater than zero and wherein x-3y=4. A pre 3,864,167 2/1975 Broadhead et al. ....... ... 136/6 LN 3,877,984 4/1975 Werth .................................. 136/6 F ferred compound is LiBS2. A preferred cell is one hav 3,925,098 12/1975 Saunders ...... -
(12) United States Patent (10) Patent No.: US 8,940,855 B2 Hedricket Al
USOO8940855B2 (12) United States Patent (10) Patent No.: US 8,940,855 B2 Hedricket al. (45) Date of Patent: Jan. 27, 2015 (54) POLYMERS BEARING PENDANT (56) References Cited PENTAFLUOROPHENYL ESTER GROUPS, AND METHODS OF SYNTHESIS AND U.S. PATENT DOCUMENTS FUNCTIONALIZATION THEREOF 3,274,214. A 9, 1966 Prochaska 4,102.912 A 7, 1978 Carr 4,515,712 A 5/1985 Wiegers et al. (71) Applicant: International Business Machines 4,613,442 A 9, 1986 Yuki et al. Corporation, Armonk, NY (US) 4,782,131 A 1 1/1988 Sweeney 4,831,100 A 5/1989 Komatsu et al. 4,888.410 A 12, 1989 Komatsu et al. (72) Inventors: James L. Hedrick, Pleasanton, CA 5,091,543 A 2/1992 Grey (US); Alshakim Nelson, Fremont, CA 5,357,027 A 10, 1994 Komatsu (US); Daniel P. Sanders, San Jose, CA 5,424.473 A 6/1995 Galvan et al. (US) 5,523,399 A 6/1996 Asaka et al. 5,869,697 A 2f1999 Bhushan et al. 6,300,458 B1 10/2001 Vandenberg (73) Assignee: International Business Machines 6,664,372 B1 12/2003 Janda et al. Corporation, Armonk, NY (US) 8,013,065 B2 9, 2011 Hedricket al. 8,044,194 B2 10/2011 Dubois et al. 8, 158,281 B2 4/2012 Ishihara et al. (*) Notice: Subject to any disclaimer, the term of this 2007, OO15932 A1 1/2007 Fujita et al. 2007/0232751 A1 10/2007 Ludewig et al. patent is extended or adjusted under 35 2007,0298.066 A1 12/2007 Alferiev et al. -
1. Active Sulfur Sites in Semimetallic Titanium Disulfide Enable CO2 Electroreduction
Research Article Cite This: ACS Catal. 2020, 10, 66−72 pubs.acs.org/acscatalysis fi Active Sulfur Sites in Semimetallic Titanium Disul de Enable CO2 Electroreduction † † ‡ # ‡ ¶ § Abdalaziz Aljabour, Halime Coskun, Xueli Zheng, , Md Golam Kibria, , Moritz Strobel, § ∥ ∥ ‡ † ⊥ ‡ Sabine Hild, Matthias Kehrer, David Stifter, Edward H. Sargent, and Philipp Stadler*, , , † § ∥ ⊥ Institute of Physical Chemistry, Institute of Polymer Science, Center for Surface and Nanoanalytics, and Linz Institute of Technology, Johannes Kepler University Linz, Altenbergerstrasse 69, 4040 Linz, Austria ‡ Edward S. Rogers Sr. Department of Electrical and Computer Engineering, University of Toronto, 10 King’s College Road, Toronto, Ontario M5S 3G4, Canada *S Supporting Information ABSTRACT: Electrocatalytic CO2-to-CO conversion repre- sents one pathway to upgrade CO2 to a feedstock for both fuels and chemicals (CO, deployed in ensuing Fischer− Tropsch or bioupgrading). It necessitates selective and energy-efficient electrocatalystsa requirement met today only using noble metals such as gold and silver. Here, we show that the two-dimensional sulfur planes in semimetallic fi titanium disul de (TiS2) provide an earth-abundant alternative. In situ Fourier transform infrared mechanistic studies reveal fi − that CO2 binds to conductive disul de planes as intermediate monothiocarbonate. The sulfur CO2 intermediate state steers fi ffi 2 the reduction kinetics toward mainly CO. Using TiS2 thin lms, we reach cathodic energy e ciencies up to 64% at 5 mA cm . We conclude with directions for the further synthesis and study of semimetallic disulfides developing CO-selective electrocatalysts. fi KEYWORDS: CO2 reduction reaction, titanium disul de, electrocatalysis ■ INTRODUCTION conductivity similar to that of graphite.29,30 We show here that TiS2 can enhance CO2 reduction because a major limitation in Electrocatalysis is an attractive pathway to recycle CO2, upgrading it using renewable energy.1 Presently, the electro- earlier used MoS2 and related materials, the poor electrical ffi conductivity, is resolved. -
To Download Program Book for This Topic in Adobe Acrobat Format
Monday Morning, October 30, 2017 Spectroscopic Ellipsometry Focus Topic are infuenced most importantly by free-electron behaviour. The obtained results are compared with those obtained by the in-situ ellipsometer. Special Room: 9 - Session EL+AS+EM+TF-MoM attention is focused on scattering of free electrons at grain boundaries and at the TiN layer interfaces. The estimated parameters are correlated with Application of SE for the Characterization of Thin Films structure changes such as grain coarsening and surface morphology. The crystallinity is analysed by X-ray Difractometry. The surface morphology of and Nanostructures the completed coatings is studied using Atomic Force Microscopy and Moderator: Tino Hofmann, University of North Carolina at Scanning Electron Microscopy. The TiN film stechiometry is estimated by Charlotte X-ray Photoemission Spectroscopy. 8:20am EL+AS+EM+TF-MoM1 Ultra-thin Plasmonic Metal Nitrides: 9:20am EL+AS+EM+TF-MoM4 Variable Temperatures Spectroscopic Optical Properties and Applications, Alexandra Boltasseva, Purdue Ellipsometry Study of the Optical Properties of InAlN/GaN Grown on University INVITED Sapphire, Y. Liang, Guangxi University, China, H.G. Gu, Huazhong Transition metal nitrides (e.g. TiN, ZrN) have emerged as promising University of Science and Technology, China, J. Xue, Xidian University, plasmonic materials due to their refractory properties and good metallic China, Chuanwei Zhang, Huazhong University of Science and Technology, properties in the visible and near infrared regions. Due to their high melting China, Q. Li, Guangxi University, China, Y. Hao, Xidian University, China, point, they may be suitable for high temperature nanophotonic applications. S.Y. Liu, Huazhong University of Science and Technology, China, Q. -
DAR-1: Guidelines for the Evaluation and Control of Ambient Air
DAR‐1 Guidelines for the Evaluation and Control of Ambient Air Contaminants Under 6NYCRR Part 212 New York State NYSDEC of Environmental Conservation DEC Program Policy Issuing Authority: Christopher Lalone Title: Director, Division of Air Resources Signature: Date Issued: Latest Date Revised: February 12, 2021 Unit: Bureau of Air Quality Analysis & Research I. SUMMARY: This policy document, issued by the New York State Department of Environmental Conservation (NYSDEC), outlines the procedures for evaluating the emissions of criteria and non-criteria air contaminants from process operations in New York State. Incorporated within the policy document are three flow charts to aid the end user when identifying applicable process emission sources, establishing uniform Environmental Ratings (ER) and ascertaining the proper degree of control for applicable process emission sources. II. POLICY: This policy is written to provide guidance for the implementation of and compliance with 6 NYCRR Part 212 Process Operations (Part 212). III. PURPOSE AND BACKGROUND: This policy provides guidance for the control of criteria and toxic air contaminants emitted from process emission sources in New York State. Process emission sources refer to the equipment at 1 manufacturing facilities that result in the release of air contaminants during operation. Process emission sources do not include equipment that combust fuel for electricity or space heating for commercial, industrial plants or residential heating. The policy describes the Division of Air Resources’ (DAR) procedures for implementing Part 212. This policy replaces the DAR-1 previously issued on August 10, 2016 by DAR. This document provides guidance to NYSDEC staff, those facility owners subject to Part 212, and the general public. -
Creating Value from Non-Carbon 2D Materials
Creating Value from Non-carbon 2D Materials - Beyond Graphene A state of the art review Contact Graphene and Other 2D Materials SIG www.ktn-uk.co.uk/interests/graphene-and-other-2d-materials Table of contents Executive Summary 5 Glossary of Non-Carbon 2D Materials 7 1. Introduction 8 2. Family of Non-Carbon 2D Materials 11 3. Synthesis and Production of Non-Carbon 2D Materials 15 4. The Innovation Landscape for Non-Carbon 2D Materials 19 5. Strategic Insights for the UK 35 6. Conclusions 42 7. Priorities and Recommendations 43 Acknowledgements 44 References 45 Appendix 1: Examples of R&D and Innovations 46 Appendix 2: Patent Analysis 48 3 4 Martin Good / Shutterstock.com Executive Summary The ability to isolate or grow two-dimensional with specially designed electrical, magnetic, (2D) materials has been a source of scientific piezoelectric and optical functionalities. fascination ever since it was shown to be possible, Research in non-carbon 2D materials has with the isolation of graphene from graphite, benefited from the earlier investments in in 2004 at the University of Manchester. The developing graphene. They can be manufactured increasing range of 2D materials extends far in a similar way and can be combined with beyond graphene and its carbon analogues. It is graphene to address its lack of band gap. Band believed that over five hundred 2D materials have gap is a property that makes silicon and other been developed globally so far. These materials semiconductors so useful for digital electronics. exhibit an exciting range of novel properties, 2D semiconducting materials are likely to become opening the door to a multitude of new potential an attractive choice for constructing digital applications. -
LTS Research Laboratories, Inc. Safety Data Sheet Hafnium Sulfide –––––––––––––––––––– 1
LTS Research Laboratories, Inc. Safety Data Sheet Hafnium Sulfide ––––––––––––––––––––––––––––––––––––––––––––––––––––––––––––––––––––––––––––––––––––––––––––– 1. Product and Company Identification ––––––––––––––––––––––––––––––––––––––––––––––––––––––––––––––––––––––––––––––––––––––––––––– Trade Name: Hafnium Sulfide Chemical Formula: HfS2 Recommended Use: Scientific research and development Manufacturer/Supplier: LTS Research Laboratories, Inc. Street: 37 Ramland Road City: Orangeburg State: New York Zip Code: 10962 Country: USA Tel #: 855-587-2436 / 855-lts-chem 24-Hour Emergency Contact: 800-424-9300 (US & Canada) +1-703-527-3887 (International) ––––––––––––––––––––––––––––––––––––––––––––––––––––––––––––––––––––––––––––––––––––––––––––– 2. Hazards Identification ––––––––––––––––––––––––––––––––––––––––––––––––––––––––––––––––––––––––––––––––––––––––––––– Signal Word: Warning Hazard Statements: H315: Causes skin irritation H319: Causes serious eye irritation H335: May cause respiratory irritation Precautionary Statements: P261: Avoid breathing dust/fume/vapor P262: Do not get in eyes, on skin, or on clothing P280: Wear protective gloves/protective clothing/eye protection/face protection P305+P351+P338: IF IN EYES: Rinse cautiously with water for several minutes. Remove contact lenses if present and easy to do – continue rinsing P304+P340: IF INHALED: Remove victim to fresh air and keep at rest in a position comfortable for breathing P403+P233: Store in a well-ventilated place. Keep container tightly closed P501: Dispose of contents/container -
ICAMD2019 Poster Presentation Schedule
ICAMD2019 Poster Presentation Schedule · Set-up : December 10 (Tue.) - December 12 (Thu.) 12:00-13:00 · Presentation Code : Presentation Date + Abstract Code (ex: PO -2D19-001 → TUE -2D19-001) · Tear-Down : December 10 (Tue.) - December 11 (Wed.) 18:00-20:30 · Presentation : December 10 (Tue.) - December 11 (Wed.) 18:00-20:00 December 12 (Thu) 18:00-19:00 December 12 (Thu) 16:00-18:00 # Session Presentation Code Presenter's Name Presenter's Affiliation Title Date & Time Place The study of artificial ultrasensitive synapses based on 2D material 1 2D + vdW Nano TUE-2D19-003 Mi Jung Lee Konkuk University Dec. 10 (Tue.) 18:00~20:00 8F Lobby CrPS4 Electrical and magnetic properties of graphene/graphene oxide 2 2D + vdW Nano TUE-2D19-005 Eun Hee Kee Konkuk University Dec. 10 (Tue.) 18:00~20:00 8F Lobby heterostructure device 3 2D + vdW Nano TUE-2D19-006 Minjeong Shin Konkuk University MoS2 field-effect transistor using CrPS4 gate insulator Dec. 10 (Tue.) 18:00~20:00 8F Lobby Room-temperature trion modulation in a light emitting van der 4 2D + vdW Nano TUE-2D19-012 Huije Ryu Seoul National University Dec. 10 (Tue.) 18:00~20:00 8F Lobby Waals heterostructure tunnel device Two dimensional ferromagnetic CrPbTe3 monolayer and strain 5 2D + vdW Nano TUE-2D19-016 imran khan Pukyong National University modulations of its magnetocrystalline anisotropy and Curie Dec. 10 (Tue.) 18:00~20:00 8F Lobby temperature Comparison magnetic properties of MoS2 and MoOx layer fabricated 6 2D + vdW Nano TUE-2D19-023 DaYea Oh Konkuk University Dec. -
Synthesis of 2D Transition Metal Oxides Robert Michael Loh, Material Science and Engineering Mentor: Prof
Synthesis of 2D Transition Metal Oxides Robert Michael Loh, Material Science and Engineering Mentor: Prof. Qing Hua Wang , Suneet Kale School for Engineering of Matter, Transport and Energy Abstract Exfoliation of HfS2 and PdSe2 CVD Synthesis Steps HfS2 • The goal of this FURI project is to synthesize thin film transition metal oxides. Metal oxides have known bulk properties but some have not been synthesized in a thin film forms. Materials take on vastly different properties when they are on the nano-scale. • Nanomaterials have been utilized in many ways such as batteries, insulation, medicine, transportation, and optics[1]. • The hypothesis of this project is that thin film transition metal oxides of germanium dioxide (GeO2), hafnium dioxide (HfO2), and palladium dioxide (PdO2) can be synthesized based on [2] previous research by the Wang research group . Their results show that molybdenum [5] trioxide (MoO3) and tungsten trioxide (WO3) can be synthesized from their respective sulfides MoS2 and WS2 by atmospheric plasma treatment in the form of thin-flake nanomaterials. First Semester Results Synthesis of HfS2 via Chemical Vapor Deposition Synthesis of GeS2 Flakes • Hafnium disulfide was also made using a method called chemical vapor deposition. • Referencing an article on material synthesis of thin flakes[3] mechanical exfoliation of A mixture of argon ( 50 sccm) and hydrogen (15 sccm) gas are passed into a tube GeS2 was found to be most favorable by plasma treating substrates to be hydrophilic furnace made of quarts at ambient pressure. They carry vaporized hafnium chloride and exposing samples to 100 oC heat for one minute before cleaving.