This Is an Open Access Document Downloaded from ORCA, Cardiff University's Institutional Repository
Total Page:16
File Type:pdf, Size:1020Kb
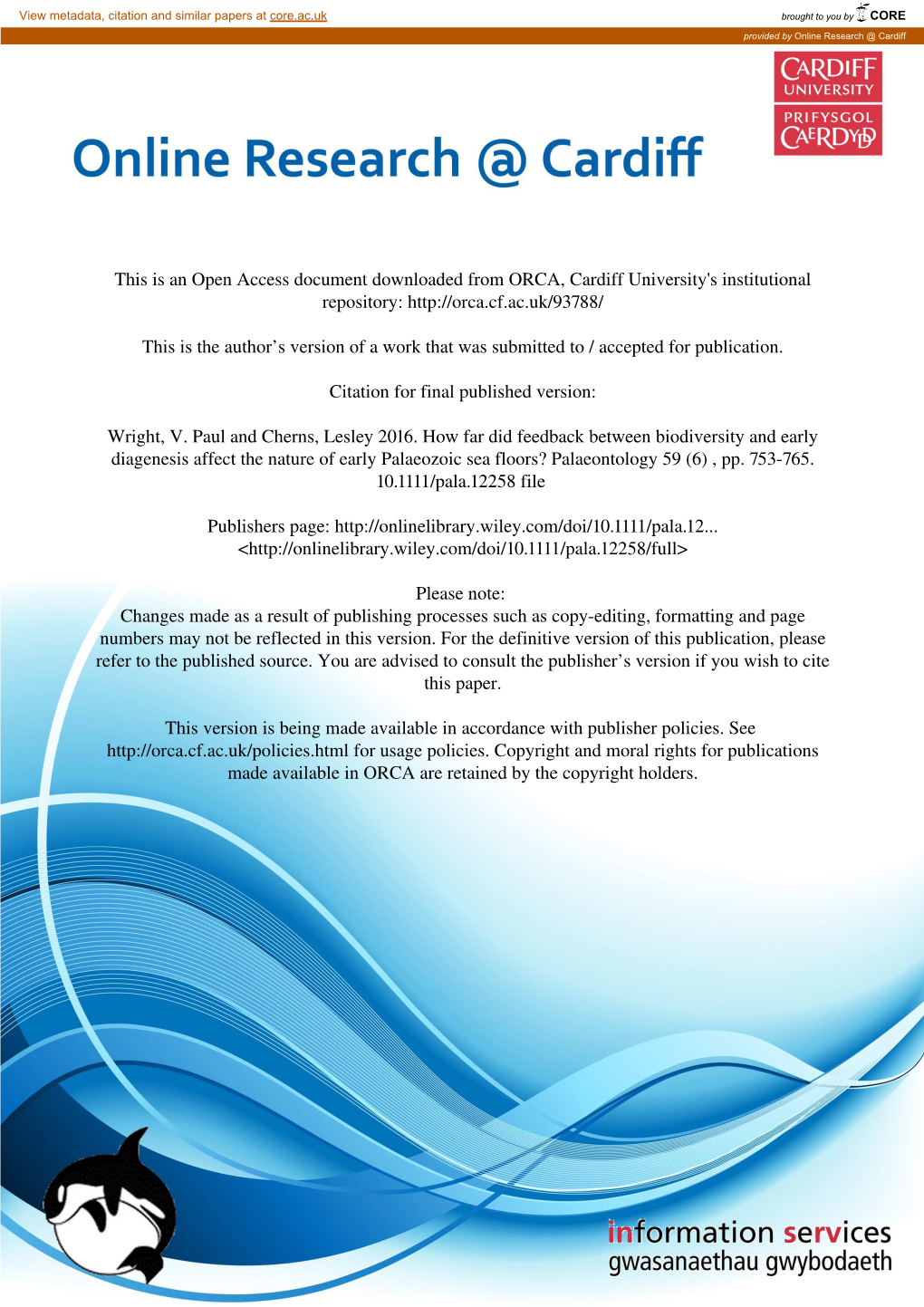
Load more
Recommended publications
-
IODP-ICDP Kolloquium 2006 in Greifswald Ernst-Moritz-Arndt-Universität
IODP-ICDP Kolloquium 2006 in Greifswald Ernst-Moritz-Arndt-Universität 27. - 29. März 2006 IODP-ICDP-Kolloquium 2006 27. - 29. März 2006 im Rahmen des 550-jährigen Jubiläums der Ernst-Moritz-Arndt-Universität Greifswald Gemeinsames Kolloquium der DFG-Schwerpunkte IODP - Integrated Ocean Drilling Program und ICDP - International Continental Scientific Drilling Program 27. - 29. März 2006 im Berufsbildungszentrum Greifswald Institut für Geographie und Geologie Friedrich-Ludwig-Jahn-Str. 17a D-17487 Greifswald Tel. 03834 864560 Email: [email protected] IODP-ICDP-Kolloquium Greifswald 27.-29.3.2006 Umgebung Tagungszentrum 500 m . r t S - u a n e h t Friedrich-Ludwig -Jahn-Str a . bft-Tankstelle R - Wolgaster Straße r Geol. e h t l Institut a P W e ß a r Tagungszentrum t S 1: Cafeteria, Icebreaker r e Karl-Liebknecht-Ring u 2: Vortragshalle 3 a h 3: Posterraum c s r (4: Schwimmbad) a Eingang 2 W Pappelallee P Geographie 1 4 Eingang Geologie F.-L.-J.-Str. 17a Makarenkostr. Parkplätze Eingang Geologenkeller Innenstadtplan Ryck Hansering Hansering Roßmühlenstraße Hörsaal Abendvortrag Straße Friedrich-Löffler-Straße J-S-Bach- Knopfstraße Brüggstraße Fischstraße Kuhstraße Steinbeckerstraße Schuhhagen Lange Straße Markt Mensa I Rathaus Dom Mühlenstraße Straße Hansering Domstraße Wolgaster Straße Wall Goethestraße Anklamer Straße Wall Martin-Luther- Stephanistraße Bahnhofstraße 200 m IODP-ICDP-Kolloquium Greifswald 27.-29.3.2006 - Programm 1 Montag, 27. März 2006 11:00 13:00 Registrierung 13:00 14:00 Eröffnung - Oberbürgermeister der Universitäts- und Hansestadt Greifswald, Dr. Arthur König - Prodekan der Mathematisch-Naturwissenschaftlichen Fakultät der Universität Greifswald, Prof. Dr. Reinhard Zölitz-Möller - DFG-Referent Dr. -
The Paleoecology and Biogeography of Ordovician Edrioasteroids
University of Tennessee, Knoxville TRACE: Tennessee Research and Creative Exchange Doctoral Dissertations Graduate School 8-2011 The Paleoecology and Biogeography of Ordovician Edrioasteroids Rene Anne Lewis University of Tennessee - Knoxville, [email protected] Follow this and additional works at: https://trace.tennessee.edu/utk_graddiss Part of the Paleontology Commons Recommended Citation Lewis, Rene Anne, "The Paleoecology and Biogeography of Ordovician Edrioasteroids. " PhD diss., University of Tennessee, 2011. https://trace.tennessee.edu/utk_graddiss/1094 This Dissertation is brought to you for free and open access by the Graduate School at TRACE: Tennessee Research and Creative Exchange. It has been accepted for inclusion in Doctoral Dissertations by an authorized administrator of TRACE: Tennessee Research and Creative Exchange. For more information, please contact [email protected]. To the Graduate Council: I am submitting herewith a dissertation written by Rene Anne Lewis entitled "The Paleoecology and Biogeography of Ordovician Edrioasteroids." I have examined the final electronic copy of this dissertation for form and content and recommend that it be accepted in partial fulfillment of the requirements for the degree of Doctor of Philosophy, with a major in Geology. Michael L. McKinney, Major Professor We have read this dissertation and recommend its acceptance: Colin D. Sumrall, Linda C. Kah, Arthur C. Echternacht Accepted for the Council: Carolyn R. Hodges Vice Provost and Dean of the Graduate School (Original signatures are on file with official studentecor r ds.) THE PALEOECOLOGY AND BIOGEOGRAPHY OF ORDOVICIAN EDRIOASTEROIDS A Dissertation Presented for the Doctor of Philosophy Degree The University of Tennessee, Knoxville René Anne Lewis August 2011 Copyright © 2011 by René Anne Lewis All rights reserved. -
This Is an Open Access Document Downloaded from ORCA, Cardiff University's Institutional Repository
This is an Open Access document downloaded from ORCA, Cardiff University's institutional repository: http://orca.cf.ac.uk/93788/ This is the author’s version of a work that was submitted to / accepted for publication. Citation for final published version: Wright, V. Paul and Cherns, Lesley 2016. How far did feedback between biodiversity and early diagenesis affect the nature of early Palaeozoic sea floors? Palaeontology 59 (6) , pp. 753-765. 10.1111/pala.12258 file Publishers page: https://doi.org/10.1111/pala.12258 <https://doi.org/10.1111/pala.12258> Please note: Changes made as a result of publishing processes such as copy-editing, formatting and page numbers may not be reflected in this version. For the definitive version of this publication, please refer to the published source. You are advised to consult the publisher’s version if you wish to cite this paper. This version is being made available in accordance with publisher policies. See http://orca.cf.ac.uk/policies.html for usage policies. Copyright and moral rights for publications made available in ORCA are retained by the copyright holders. SYMPOSIUM How far did feedback between biodiversity and early diagenesis affect the nature of early Palaeozoic sea floors? By V. PAUL WRIGHT1 and LESLEY CHERNS2 1 Natural Sciences, National Museum of Wales, Cathays Park, Cardiff, CF10 3NP, UK; e- mail [email protected] 2 School of Earth and Ocean Sciences, Cardiff University, Main Building, Park Place, Cardiff CF10 3AT, UK; e-mail [email protected] Abstract: Latest Precambrian to Early Palaeozoic biosphere evolution triggered changes in early diagenesis and carbonate precipitation which fed back to biodiversity through colonisation of hard substrates. -
Sea Level, Carbonate Mineralogy, and Early Diagenesis Controlled Δ13c Records in Upper Ordovician Carbonates David S
https://doi.org/10.1130/G46861.1 Manuscript received 10 August 2019 Revised manuscript received 17 October 2019 Manuscript accepted 29 October 2019 © 2019 The Authors. Gold Open Access: This paper is published under the terms of the CC-BY license. Published online 9 December 2019 Sea level, carbonate mineralogy, and early diagenesis controlled δ13C records in Upper Ordovician carbonates David S. Jones1, R. William Brothers1, Anne-Sofe Crüger Ahm2, Nicholas Slater2, John A. Higgins2 and David A. Fike3 1 Geology Department, Amherst College, 11 Barrett Hill Road, Amherst, Massachusetts 01002, USA 2 Department of Geosciences, Princeton University, Guyot Hall, Princeton, New Jersey 08544, USA 3 Department of Earth & Planetary Sciences, Washington University in St. Louis, 1 Brookings Drive, St. Louis, Missouri 63130, USA ABSTRACT (Melchin and Holmden, 2006). However, these Stratigraphic variability in the geochemistry of sedimentary rocks provides critical data models can require unrealistic changes to carbon for interpreting paleoenvironmental change throughout Earth history. However, the vast burial and/or weathering fuxes, or they predict majority of pre-Jurassic geochemical records derive from shallow-water carbonate platforms cross-platform δ13C gradients (δ13C increasing that may not refect global ocean chemistry. Here, we used calcium isotope ratios (δ44Ca) in with greater proximity to the coast) that con- conjunction with minor-element geochemistry (Sr/Ca) and feld observations to explore the tradict the variability observed in some -
Bryozoan Skeletal Index (BSI): a Measure of the Degree of Calcification in Stenolaemate Bryozoans
BRYOZOAN STUDIES 2019 Bryozoan Skeletal Index (BSI): a measure of the degree of calcification in stenolaemate bryozoans Patrick N. Wyse Jackson1*, Marcus M. Key, Jr.2 and Catherine M. Reid3 1 Department of Geology, Trinity College, Dublin 2, Ireland [*corresponding author: e-mail: [email protected]] 2 Department of Earth Sciences, Dickinson College, Carlisle, Pennsylvania 17013-2896, USA [e-mail: [email protected]] 3 School of Earth and Environment, University of Canterbury, Private Bag 4800, Christchurch, New Zealand [e-mail: [email protected]] ABSTRACT minimal. In this study the differences observed in The Upper Ordovician of the Cincinnati Arch region BSI between trepostome and cystoporate species in of the United States has yielded a highly diverse the Cincinnatian is significant, and ramose colonies bryozoan fauna, and which provides an excellent show a higher BSI than encrusting zoaria in the data source for use in this study that proposes same fauna. a novel measure of the degree of skeletal material in Palaeozoic stenolaemate bryozoans. This study is based on 16 trepostome species and one cystoporate INTRODUCTION species described from the Dillsboro Formation Bryozoans of the Class Stenolaemata are characterised (Maysvillian to early Richmondian, Cincinnatian) of by having autozooecial chambers that are broadly Indiana and in 20 species (15 trepostomes and five tubular in nature. They were significant members of cystoporates) from the Lexington Limestone and the Palaeozoic faunas appearing in the Ordovician Clays Ferry -
Alleged Cnidarian Sphenothallus in the Late Ordovician of Baltica, Its Mineral Composition and Microstructure
Alleged cnidarian Sphenothallus in the Late Ordovician of Baltica, its mineral composition and microstructure OLEV VINN and KALLE KIRSIMÄE Vinn, O. and Kirsimäe, K. 2015. Alleged cnidarian Sphenothallus in the Late Ordovician of Baltica, its mineral compo- sition and microstructure. Acta Palaeontologica Polonica 60 (4): 1001–1008. Sphenothallus is a problematic fossil with possible cnidarian affinities. Two species of Sphenothallus, S. aff. longissimus and S. kukersianus, occur in the normal marine sediments of the Late Ordovician of Estonia. S. longissimus is more common than S. kukersianus and has a range from early Sandbian to middle Katian. Sphenothallus had a wide paleo- biogeographic distribution in the Late Ordovician. The tubes of Sphenothallus are composed of lamellae with a homo- geneous microstructure. The homogeneous microstructure could represent a diagenetic fabric, based on the similarity to diagenetic structures in Torellella (Cnidaria?, Hyolithelminthes). Tubes of Sphenothallus have an apatitic composition, but one tube contains lamellae of diagenetic calcite within the apatitic structure. Sphenothallus presumably had origi- nally biomineralized apatitic tubes. Different lattice parameters of the apatite indicate that biomineralization systems of phosphatic cnidarians Sphenothallus and Conularia sp. may have been different. Key words: Cnidaria?, Sphenothallus, apatite, microstructure, Ordovician, Sandbian, Katian, Estonia. Olev Vinn [[email protected]] and Kalle Kirsimäe [[email protected]], Department of Geology, University of Tartu, Ravila 14A, 50411 Tartu, Estonia. Copyright © 2015 O. Vinn and K. Kirsimäe. This is an open-access article distributed under the terms of the Creative Commons Attribution License (for details please see http://creativecommons.org/licenses/by/4.0/), which permits un- restricted use, distribution, and reproduction in any medium, provided the original author and source are credited. -
Biomineralization and Evolutionary History Andrew H
1 111 Biomineralization and Evolutionary History Andrew H. Knoll Department of Organismic and Evolutionary Biology Harvard University Cambridge, Massachusetts, 02138 U.S.A. INTRODUCTION The Dutch ethologist Niko Tinbergen famously distinguished between proximal and ultimate explanations in biology. Proximally, biologists seek a mechanistic understanding of how organisms function; most of this volume addresses the molecular and physiological bases of biomineralization. But while much of biology might be viewed as a particularly interesting form of chemistry, it is more than that. Biology is chemistry with a history, requiring that proximal explanations be grounded in ultimate, or evolutionary, understanding. The physiological pathways by which organisms precipitate skeletal minerals and the forms and functions of the skeletons they fashion have been shaped by natural selection through geologic time, and all have constrained continuing evolution in skeleton-forming clades. In this chapter, I outline some major patterns of skeletal evolution inferred from phylogeny and fossils (Figure 1), highlighting ways that our improving mechanistic knowledge of biomineralization can help us to understand this evolutionary record (see Leadbetter and Riding 1986; Lowenstam and Weiner 1989; Carter 1990; and Simkiss and Wilbur 1989 for earlier reviews). Figure 1. A geologic time scale for the past 1000 million years, showing the principal time divisions used in Earth science and the timing of major evolutionary events discussed in this chapter. Earlier intervals of time—the Mesoproterozoic (1600–1000 million years ago) and Paleoproterozoic (2500– 1600 million years ago) eras of the Proterozoic Eon and the Archean Eon (> 2500 million years ago)— are not shown. Time scale after Remane (2000). -
Organism-Sediment Relationships in Silurian Marine Environments
ORGANISM-SEDIMENT RELATIONSHIPS IN SILURIAN MARINE ENVIRONMENTS by C. E. BRETT ABSTRACT.Organisms pIayed major roles as producers and modifiers of sediments in many Silurian marine env~ronments.Pelrnarozoan skeietal debris famed extensive shoals and banks in many high-enerw, tropical environments : algae. bvozoans. tabulate corals. and strematoporoids were the major skeieral contributors to reeial buridups common in mid and late SiIunan subtropical shelves. ArtlcuIare brachiopods and Lesser numben oiosrracodes, trilobites, and molluscs formed extensive. although reIatively !Em (5-15 mi. shell beds in shaIloiv shelf fac~es:pefagc. cephalopod limestones characrerized the circum-Gondwana region. Skeletd hard substrates and hardgrounds supported relarively complex eprfaunal suspenaan-feeding communities. The apparent scarclry of encollthic sponges and bivalves indicates thac rates of hard subsrrare bioerosron were far below those of post-Palaeozolc times. Substra~eselection. spatial compeotion. anc porarlry of c:Tpnc versus exposed subcommun~tiesare clearly evidenr. for the first rzme. in Ordovician to latesr Siiurian hard substrate cornrnuniries. Unconsoi~datedsediments displayed a wide variety of characrerisrtc trace and body fossd assoclatlons that were. to some degree. subsrrare conrrolled. Sand and silt subsrrates mpporred a low die'ersltv oi shelly organisms bur preserved abundant rraccs, nngmg from fkoiirhas ana .-~rthropir~cus-aomina~ed nensshore sed~mentsro offshore diverse ichnolicles. Some Silur~annearshore sediments display b~orurbat~on up to Im deep and contaln very Fen epifaunal organisms. susgesting destabiiizat~onof substrares. However. b1ogentc disturbance of offsfshore, soft substrates rematned relat~velyresrricted In the Sllur~anwnh tnfauna extenarng onlv abour 1C-15 cm tnro the sediment. Silunan epthunai organlrrns dispIayea varled morpnolo_eical ad~ptationsrs soft substrates. -
Decoupled Evolution of Soft and Hard Substrate Communities During the Cambrian Explosion and Great Ordovician Biodiversification Event
Decoupled evolution of soft and hard substrate communities during the Cambrian Explosion and Great Ordovician Biodiversification Event Luis A. Buatoisa,1, Maria G. Mánganoa, Ricardo A. Oleab, and Mark A. Wilsonc aDepartment of Geological Sciences, University of Saskatchewan, Saskatoon, SK, Canada S7N 5E2; bEastern Energy Resources Science Center, US Geological Survey, Reston, VA 20192; and cDepartment of Geology, The College of Wooster, Wooster, OH 44691 Edited by Steven M. Holland, University of Georgia, Athens, GA, and accepted by Editorial Board Member David Jablonski May 6, 2016 (received for review November 21, 2015) Contrasts between the Cambrian Explosion (CE) and the Great shed light on the natures of both radiations. Because there is still Ordovician Biodiversification Event (GOBE) have long been recog- controversy regarding Sepkoski’s nonstandardized curves of nized. Whereas the vast majority of body plans were established Phanerozoic taxonomic diversity (13–15), a rarefaction analysis as a result of the CE, taxonomic increases during the GOBE were was performed in an attempt to standardize diversity data. The manifested at lower taxonomic levels. Assessing changes of ichno- aims of this paper are to document the contrasting ichnodiversity diversity and ichnodisparity as a result of these two evolutionary and ichnodisparity trajectories in soft and hard substrate com- events may shed light on the dynamics of both radiations. The early munities during these two evolutionary events and to discuss the Cambrian (series 1 and 2) displayed a dramatic increase in ichnodi- possible underlying causes of this decoupled evolution. versity and ichnodisparity in softground communities. In contrast to this evolutionary explosion in bioturbation structures, only a few Results Cambrian bioerosion structures are known. -
The Palaeontology Newsletter
The Palaeontology Newsletter Contents 90 Editorial 2 Association Business 3 Association Meetings 11 News 14 From our correspondents Legends of Rock: Marie Stopes 22 Behind the scenes at the Museum 25 Kinds of Blue 29 R: Statistical tests Part 3 36 Rock Fossils 45 Adopt-A-Fossil 48 Ethics in Palaeontology 52 FossilBlitz 54 The Iguanodon Restaurant 56 Future meetings of other bodies 59 Meeting Reports 64 Obituary: David M. Raup 79 Grant and Bursary Reports 81 Book Reviews 103 Careering off course! 111 Palaeontology vol 58 parts 5 & 6 113–115 Papers in Palaeontology vol 1 parts 3 & 4 116 Virtual Palaeontology issues 4 & 5 117–118 Annual Meeting supplement >120 Reminder: The deadline for copy for Issue no. 91 is 8th February 2016. On the Web: <http://www.palass.org/> ISSN: 0954-9900 Newsletter 90 2 Editorial I watched the press conference for the publication on the new hominin, Homo naledi, with rising incredulity. The pomp and ceremony! The emotion! I wondered why all of these people were so invested just because it was a new fossil species of something related to us in the very recent past. What about all of the other new fossil species that are discovered every day? I can’t imagine an international media frenzy, led by deans and vice chancellors amidst a backdrop of flags and flashbulbs, over a new species of ammonite. Most other fossil discoveries and publications of taxonomy are not met with such fanfare. The Annual Meeting is a time for sharing these discoveries, many of which will not bring the scientists involved international fame, but will advance our science and push the boundaries of our knowledge and understanding. -
Shallow-Marine Carbonates
See discussions, stats, and author profiles for this publication at: https://www.researchgate.net/publication/286164562 Shallow-Marine Carbonates Article in Developments in Sedimentology · December 2012 DOI: 10.1016/B978-0-444-53813-0.00023-X CITATIONS READS 15 221 3 authors, including: D. Knaust A. V. Dronov Statoil ASA Russian Academy of Scie… 65 PUBLICATIONS 888 57 PUBLICATIONS 827 CITATIONS CITATIONS SEE PROFILE SEE PROFILE Some of the authors of this publication are also working on these related projects: Revision of the ichnogenus Arenicolites SALTER, 1857 View project Revision of the ichnogenus Teichichnus View project All content following this page was uploaded by D. Knaust on 15 October 2017. The user has requested enhancement of the downloaded file. Chapter 23 Shallow-Marine Carbonates Dirk Knaust,*,1 H. Allen Curran† and Andrei V. Dronov‡ *Statoil ASA, Stavanger, Norway, †Department of Geosciences, Smith College, Northampton, Massachusetts, USA, ‡Russian Academy of Sciences, Geological Institute, Moscow, Russia 1Corresponding author: e-mail: [email protected] 1. INTRODUCTION Carbonates comprise only 20–25% of the total volume of all sedimentary rocks (Boggs, 2009). This fact is reflected in the relatively small number of ichnolo- gical studies of carbonates compared with siliciclastics, although other aspects (such as sedimentology or diagenesis) are roughly equally covered. Given the importance of carbonates as oil and gas reservoirs and aquifers (about 50% of the world’s total proven hydrocarbon reserves are held in carbonate reservoirs), the ichnology of carbonate sedimentary systems has much potential for future investigations. This chapter deals with the ichnology of shallow-marine carbonates, ranging from marginal-marine (peritidal) environments to the shelf. -
Salt Lakes of the Northern Great Plains. Field Trip
Front Cover Supplied by LOC Title Page Supplied by LOC SALT LAKES OF THE NORTHERN GREAT PLAINS William M. Last Department of Geological Sciences University of Manitoba Winnipeg, Canada R3T 2N2 email: [email protected] and Lynn I. Kelley Saskatchewan Geological Survey Saskatchewan Energy and Mines Regina, Canada S4P 3V7 email: [email protected] Post-Conference Field Trip B2 May 30-31, 2002 SALT LAKES OF THE NORTHERN GREAT PLAINS Table of Contents 1. WELCOME & OBJECTIVES OF EXCURSION.......................................................................1 1.1 ACKNOWLEDGMENTS.............................................................................................2 2. ITINERARY & GENERAL ADVICE TO PARTICIPANTS.....................................................3 2.1 THURSDAY, MAY 30.................................................................................................3 2.2 FRIDAY, MAY 31........................................................................................................4 2.3 MISCELLANEOUS ADVICE......................................................................................4 3. INTRODUCTORY COMMENT: THE NORTHERN GREAT PLAINS...................................5 3.1 INTRODUCTION.........................................................................................................5 3.2 SCOPE & RATIONALE OF GEOLIMNOLOGY IN THE NORTHERN GREAT PLAINS...................................................................................................................5 3.3 SETTING & PHYSICAL BACKGROUND