Identification and Characterisation of Hypomethylated DNA Loci Controlling Quantitative Resistance in Arabidopsis
Total Page:16
File Type:pdf, Size:1020Kb
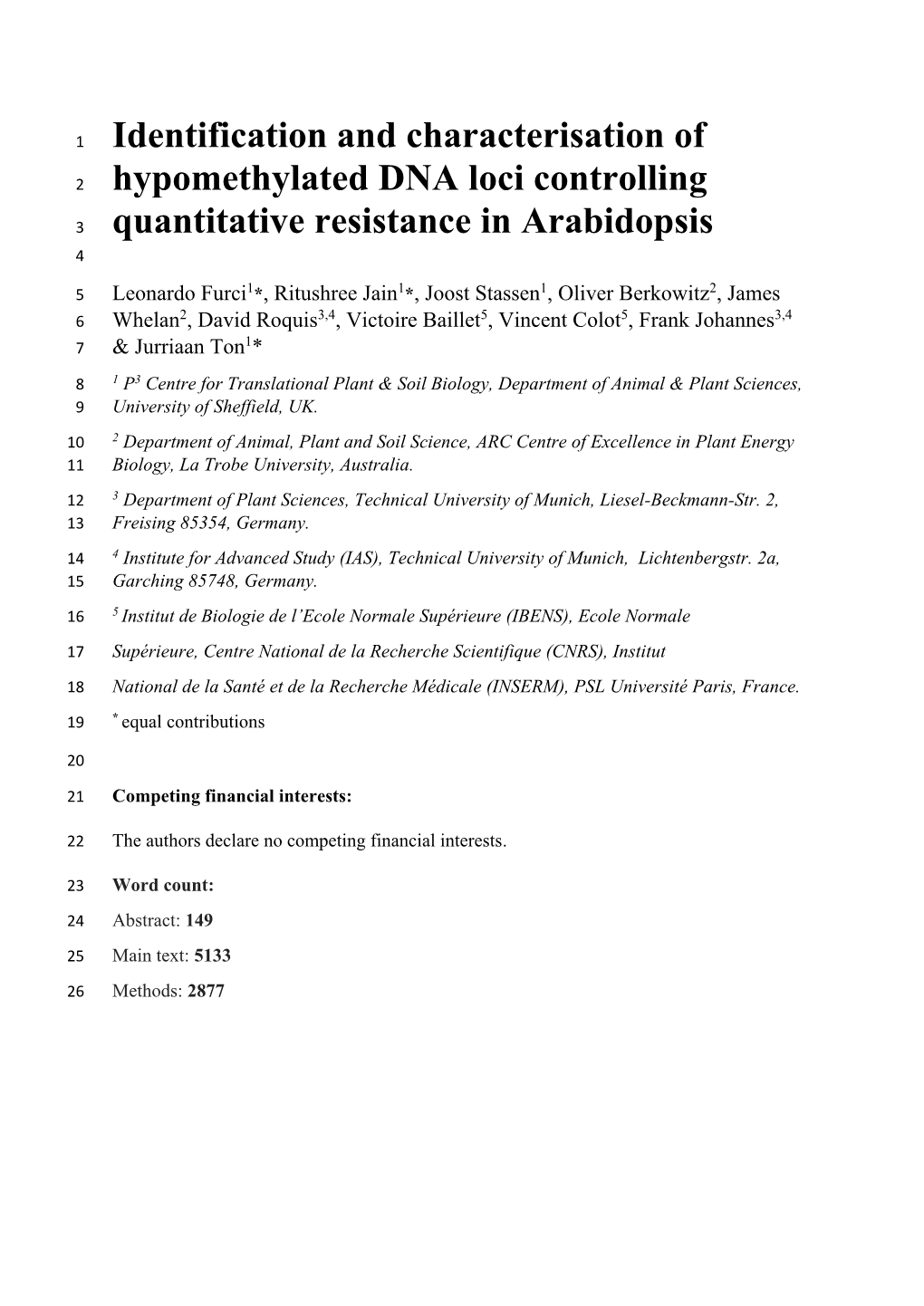
Load more
Recommended publications
-
Critical Evaluation of Gene Expression Changes in Human Tissues In
Review Critical Evaluation of Gene Expression Changes in Human Tissues in Response to Supplementation with Dietary Bioactive Compounds: Moving Towards Better-Quality Studies Biljana Pokimica 1 and María-Teresa García-Conesa 2,* 1 Center of Research Excellence in Nutrition and Metabolism, Institute for Medical Research, University of Belgrade, Belgrade 11000, Serbia; [email protected] 2 Research Group on Quality, Safety and Bioactivity of Plant Foods, Campus de Espinardo, Centro de Edafologia y Biologia Aplicada del Segura-Consejo Superior de Investigaciones Científicas (CEBAS-CSIC), P.O. Box 164, 30100 Murcia, Spain * Correspondence: [email protected]; Tel.: +34-968-396276 Received: 4 June 2018; Accepted: 19 June 2018; Published: 22 June 2018 Abstract: Pre-clinical cell and animal nutrigenomic studies have long suggested the modulation of the transcription of multiple gene targets in cells and tissues as a potential molecular mechanism of action underlying the beneficial effects attributed to plant-derived bioactive compounds. To try to demonstrate these molecular effects in humans, a considerable number of clinical trials have now explored the changes in the expression levels of selected genes in various human cell and tissue samples following intervention with different dietary sources of bioactive compounds. In this review, we have compiled a total of 75 human studies exploring gene expression changes using quantitative reverse transcription PCR (RT-qPCR). We have critically appraised the study design and methodology used as well as the gene expression results reported. We herein pinpoint some of the main drawbacks and gaps in the experimental strategies applied, as well as the high interindividual variability of the results and the limited evidence supporting some of the investigated genes as potential responsive targets. -
A Natural Antimicrobial Ingredient
Mustard: A Natural Antimicrobial Ingredient Did you know? Mustard has natural antimicrobial properties, the bioactive compounds ‐ glucosinolates in mustard, are converted to the antimicrobial isothiocyanates in the presence of water Natural preservative functionality of mustard can be very valuable to the food industry Mustard isothiocyanates can effect up to a 5‐log reduction of E. coli 0157:H7 in fermented meats Mustard Essential Oils (MEO) can be added to bakery products to inhibit fungal growth and production of aflatoxins Glucosinolates from deheated / deodorized (bland) mustard can be converted into highly antimicrobial isothiocyanate by bacterial myrosinase‐like enzyme action present in E. coli, 0157:H7, Staphylococcus carnosus and Pediococcus pentosaceus11,12,13 and in L. monocytogenes, Enterococcus faecalis, Staphylococcus aureus and Salmonella typhimurium Mustard’s inherent antimicrobial properties should fit well with the food industry’s growing interest and increasing consumer demand for the use of a natural preservative to enhance food safety and increase shelf‐life of prepared packaged foods with a “clean label” claim. Mustards in Foods Mustards (Yellow and Brown) are commercially available as whole seeds, ground/cracked seeds, meals or flour forms and are widely used in the manufacture of condiments, salad dressings, pickles, sauces, processed meats and as substitutes for egg ingredients. While mainly used as a spice or for its functional properties, mustard can also provide raw and processed foods protection against pathogenic and spoilage microorganisms. Antimicrobial Bioactives in Mustard All mustards, Yellow (& White) (Sinapis alba) and Brown/Oriental (Brassica juncea), contain glucosinolates. It is these glucosinolates and their isothiocyanate (ITC) breakdown products which contribute to its natural antimicrobial activity and to the heat and pungency of mustard. -
THE Glucosinolates & Cyanogenic Glycosides
THE Glucosinolates & Cyanogenic Glycosides Assimilatory Sulphate Reduction - Animals depend on organo-sulphur - In contrast, plants and other organisms (e.g. fungi, bacteria) can assimilate it - Sulphate is assimilated from the environment, reduced inside the cell, and fixed to sulphur containing amino acids and other organic compounds Assimilatory Sulphate Reduction The Glucosinolates The Glucosinolates - Found in the Capparales order and are the main secondary metabolites in cruciferous crops The Glucosinolates - The glucosinolates are a class of organic compounds (water soluble anions) that contain sulfur, nitrogen and a group derived from glucose - Every glucosinolate contains a central carbon atom which is bond via a sulfur atom to the glycone group, and via a nitrogen atom to a sulfonated oxime group. In addition, the central carbon is bond to a side group; different glucosinolates have different side groups The Glucosinolates Central carbon atom The Glucosinolates - About 120 different glucosinolates are known to occur naturally in plants. - They are synthesized from certain amino acids: methionine, phenylalanine, tyrosine or tryptophan. - The plants contain the enzyme myrosinase which, in the presence of water, cleaves off the glucose group from a glucosinolate The Glucosinolates -Post myrosinase activity the remaining molecule then quickly converts to a thiocyanate, an isothiocyanate or a nitrile; these are the active substances that serve as defense for the plant - To prevent damage to the plant itself, the myrosinase and glucosinolates -
PYK10 Myrosinase Reveals a Functional Coordination Between Endoplasmic Reticulum Bodies and Glucosinolates in Arabidopsis Thaliana
The Plant Journal (2017) 89, 204–220 doi: 10.1111/tpj.13377 PYK10 myrosinase reveals a functional coordination between endoplasmic reticulum bodies and glucosinolates in Arabidopsis thaliana Ryohei T. Nakano1,2,3, Mariola Pislewska-Bednarek 4, Kenji Yamada5,†, Patrick P. Edger6,‡, Mado Miyahara3,§, Maki Kondo5, Christoph Bottcher€ 7,¶, Masashi Mori8, Mikio Nishimura5, Paul Schulze-Lefert1,2,*, Ikuko Hara-Nishimura3,*,#,k and Paweł Bednarek4,*,# 1Department of Plant Microbe Interactions, Max Planck Institute for Plant Breeding Research, Carl-von-Linne-Weg 10, D-50829 Koln,€ Germany, 2Cluster of Excellence on Plant Sciences (CEPLAS), Max Planck Institute for Plant Breeding Research, Carl-von-Linne-Weg 10, D-50829 Koln,€ Germany, 3Department of Botany, Graduate School of Science, Kyoto University, Sakyo-ku, Kyoto 606-8502, Japan, 4Institute of Bioorganic Chemistry, Polish Academy of Sciences, Noskowskiego 12/14, 61-704 Poznan, Poland, 5Department of Cell Biology, National Institute of Basic Biology, Okazaki 444-8585, Japan, 6Department of Plant and Microbial Biology, University of California, Berkeley, CA 94720, USA, 7Department of Stress and Developmental Biology, Leibniz Institute of Plant Biochemistry, D-06120 Halle (Saale), Germany, and 8Ishikawa Prefectural University, Nonoichi, Ishikawa 834-1213, Japan Received 29 March 2016; revised 30 August 2016; accepted 5 September 2016; published online 19 December 2016. *For correspondence (e-mails [email protected]; [email protected]; [email protected]). #These authors contributed equally to this work. †Present address: Malopolska Centre of Biotechnology, Jagiellonian University, 30-387 Krakow, Poland. ‡Present address: Department of Horticulture, Michigan State University, East Lansing, MI, USA. §Present address: Department of Biological Sciences, Graduate School of Science, The University of Tokyo, Tokyo 113-0033, Japan. -
Opposing Effects of Glucosinolates on a Specialist Herbivore and Its Predators
Journal of Applied Ecology 2011, 48, 880–887 doi: 10.1111/j.1365-2664.2011.01990.x Chemically mediated tritrophic interactions: opposing effects of glucosinolates on a specialist herbivore and its predators Rebecca Chaplin-Kramer1*, Daniel J. Kliebenstein2, Andrea Chiem3, Elizabeth Morrill1, Nicholas J. Mills1 and Claire Kremen1 1Department of Environmental Science Policy & Management, University of California, Berkeley, 130 Mulford Hall #3114, Berkeley, CA 94720, USA; 2Department of Plant Sciences, University of California, Davis, One Shields Ave., Davis, CA 95616, USA; and 3Department of Integrative Biology, University of California, Berkeley, 3060 Valley Life Sciences Bldg #3140, Berkeley, CA 94720, USA Summary 1. The occurrence of enemy-free space presents a challenge to the top-down control of agricultural pests by natural enemies, making bottom-up factors such as phytochemistry and plant distributions important considerations for successful pest management. Specialist herbivores like the cabbage aphid Brevicoryne brassicae co-opt the defence system of plants in the family Brassicaceae by sequestering glucosinolates to utilize in their own defence. The wild mustard Brassica nigra,analter- nate host for cabbage aphids, contains more glucosinolates than cultivated Brassica oleracea,and these co-occur in agricultural landscapes. We examined trade-offs between aphid performance and predator impact on these two host plants to test for chemically mediated enemy-free space. 2. Glucosinolate content of broccoli B. oleracea and mustard B. nigra was measured in plant mat- ter and in cabbage aphids feeding on each food source. Aphid development, aphid fecundity, preda- tion and predator mortality, and field densities of aphids and their natural enemies were also tested for each food source. -
Molecular Mechanisms of Fibroblast Growth Factor Signaling in Physiology and Pathology
Downloaded from http://cshperspectives.cshlp.org/ on September 28, 2021 - Published by Cold Spring Harbor Laboratory Press Molecular Mechanisms of Fibroblast Growth Factor Signaling in Physiology and Pathology Artur A. Belov and Moosa Mohammadi Department of Biochemistry and Molecular Pharmacology, New York University School of Medicine, New York, New York 10016 Correspondence: [email protected] Fibroblast growth factors (FGFs) signal in a paracrine or endocrine fashion to mediate a myriad of biological activities, ranging from issuing developmental cues, maintaining tissue homeostasis, and regulating metabolic processes. FGFs carry out their diverse func- tions by binding and dimerizing FGF receptors (FGFRs) in a heparan sulfate (HS) cofactor- or Klotho coreceptor-assisted manner. The accumulated wealth of structural and biophysical data in the past decade has transformed our understanding of the mechanism of FGF signal- ing in human health and development, and has provided novel concepts in receptor tyrosine kinase (RTK) signaling. Among these contributions are the elucidation of HS-assisted recep- tor dimerization, delineation of the molecular determinants of ligand–receptor specificity, tyrosine kinase regulation, receptor cis-autoinhibition, and tyrosine trans-autophosphoryla- tion. These structural studies have also revealed how disease-associated mutations highjack the physiological mechanisms of FGFR regulation to contribute to human diseases. In this paper, we will discuss the structurally and biophysically derived mechanisms of FGF signal- ing, and how the insights gained may guide the development of therapies for treatment of a diverse array of human diseases. ibroblast growth factor (FGF) signaling ful- (Goldfarb 1996; Kato and Sekine 1999; Sekine Ffills essential roles in metazoan development et al. -
An Introduction to Nutrition and Metabolism, 3Rd Edition
INTRODUCTION TO NUTRITION AND METABOLISM INTRODUCTION TO NUTRITION AND METABOLISM third edition DAVID A BENDER Senior Lecturer in Biochemistry University College London First published 2002 by Taylor & Francis 11 New Fetter Lane, London EC4P 4EE Simultaneously published in the USA and Canada by Taylor & Francis Inc 29 West 35th Street, New York, NY 10001 Taylor & Francis is an imprint of the Taylor & Francis Group This edition published in the Taylor & Francis e-Library, 2004. © 2002 David A Bender All rights reserved. No part of this book may be reprinted or reproduced or utilised in any form or by any electronic, mechanical, or other means, now known or hereafter invented, including photocopying and recording, or in any information storage or retrieval system, without permission in writing from the publishers. British Library Cataloguing in Publication Data A catalogue record for this book is available from the British Library Library of Congress Cataloging in Publication Data Bender, David A. Introduction to nutrition and metabolism/David A. Bender.–3rd ed. p. cm. Includes bibliographical references and index. 1. Nutrition. 2. Metabolism. I. Title. QP141 .B38 2002 612.3′9–dc21 2001052290 ISBN 0-203-36154-7 Master e-book ISBN ISBN 0-203-37411-8 (Adobe eReader Format) ISBN 0–415–25798–0 (hbk) ISBN 0–415–25799–9 (pbk) Contents Preface viii Additional resources x chapter 1 Why eat? 1 1.1 The need for energy 2 1.2 Metabolic fuels 4 1.3 Hunger and appetite 6 chapter 2Enzymes and metabolic pathways 15 2.1 Chemical reactions: breaking and -
DNA Damage, Repair and Mutational Spectrum
University of Rhode Island DigitalCommons@URI Open Access Dissertations 2019 DNA damage, repair and mutational spectrum Ke Bian University of Rhode Island, [email protected] Follow this and additional works at: https://digitalcommons.uri.edu/oa_diss Recommended Citation Bian, Ke, "DNA damage, repair and mutational spectrum" (2019). Open Access Dissertations. Paper 850. https://digitalcommons.uri.edu/oa_diss/850 This Dissertation is brought to you for free and open access by DigitalCommons@URI. It has been accepted for inclusion in Open Access Dissertations by an authorized administrator of DigitalCommons@URI. For more information, please contact [email protected]. DNA DAMAGE, REPAIR, AND MUTATIONAL SPECTRUM BY KE BIAN A DISSERTATION SUBMITTED IN PARTIAL FULFILLMENT OF THE REQUIREMENTS FOR THE DEGREE OF DOCTOR OF PHILOSOPHY IN PHARMACEUTICAL SCIENCES UNIVERSITY OF RHODE ISLAND 2019 DOCTOR OF PHILOSOPHY DISSERTATION OF KE BIAN APPROVED: Dissertation Committee: Major Professor Deyu Li Bongsup Cho Gongqin Sun Nasser H. Zawia DEAN OF THE GRADUATE SCHOOL UNIVERSITY OF RHODE ISLAND 2019 ABSTRACT The integrity and stability of DNA is essential to life since it stores genetic information in every living cell. Chemicals from the environment will assault DNA to form various types of DNA damage, ranging from small covalent crosslinks between neighboring DNA bases as seen in cyclobutane pyrimidine dimers, to big bulky adducts derived from benzo[a]pyrene. This resultant damage will lead to replication block and mutation if remain unrepaired and will eventually cause cancer or other genetic diseases. The work presented in this dissertation has illustrated the important role of the AlkB family DNA repair enzymes in cancer and Wilson’s Disease. -
Project No 10 the Insect Mustard Oil Bomb
Project no 10 The insect mustard oil bomb: a chemical weapon against predators and pathogens? Supervisors: Dr. Franziska Beran Research Group Detoxification and Sequestration in Insects, Max Planck Institute for Chemical Ecology Dr. Hannah Rowland Research Group Predators and Prey, Max Planck Institute for Chemical Ecology Background: Plants and animals are well-known to use chemical weapons to defend themselves against enemies. When this chemical defence is produced by the organism itself, a major challenge is to store the toxin safely, but ready for action when needed. Plants have solved this problem by developing two-component defence systems (1). The best studied example is the so-called 'mustard- oil bomb' in crucifers, where high amounts of non-toxic mustard-oil glucosides (glucosinolates) are stored in all plant tissues but separately from a specific β-thioglucosidase enzyme known as myrosinase (2). When the plant tissue is damaged by herbivore feeding, mustard oil glucosides are rapidly degraded to toxic mustard oils (isothiocyanates). These are responsible for the sharp taste of mustard and wasabi. Due to their high reactivity, mustard oils have broad activity against bacteria, fungi, nematodes, and small herbivores. We have previously shown that Phyllotreta flea beetles emit toxic mustard-oils, and that this emission is independent from mustard-oil glucosides in the current food plant (3). We discovered that Phyllotreta beetles accumulate and store mustard-oil glucosides in their body up to a level of 2% of their body weight. The beetles also produce their own myrosinase enzyme (4). Furthermore, recent results revealed that all life stages of Phyllotreta contain mustard oil glucosides, and that high myrosinase activity is present in both larvae and adults. -
The Metabolism of Plant Glucosinolates by Gut Bacteria
The metabolism of plant glucosinolates by gut bacteria Fatma Cebeci A thesis submitted for the degree of Doctor of Philosophy to the University of East Anglia Institute of Food Research April, 2017 © This copy of the thesis has been supplied on condition that anyone who consults it is understood to recognise that its copyright rests with the author and that use of any information derived there from must be in accordance with current UK Copyright Law. In addition, any quotation or extract must include full attribution. PhD Thesis 2017 Fatma Cebeci Metabolism of Plant Glucosinolates by Gut Bacteria ABSTRACT Glucosinolates found in cruciferous vegetables are degraded by plant myrosinases into bioactive isothiocyanates (ITCs) which have been recognised as potent anticancer compounds. During cooking, plant myrosinases are heat inactivated so ITC production is dependent on the myrosinase-like enzymes produced by the gut bacteria. This study is focused on investigating glucosinolate metabolism by the human gut bacteria and identifying the enzymes that play a crucial role. Human gut bacteria that were previously reported to metabolise glucosinolates were investigated in this study. In addition, 98 more human gut strains were isolated using a glucoraphanin enrichment method. It was hypothesised that bacterial myrosinases are β- glucosidases with specificity for glucosinolates. To identify the first bacterial myrosinase from the human gut, four putative β-glucosidases from Enterococcus casseliflavus CP1 and Escherichia coli FI10944 were cloned and heterologously expressed in E. coli. An alternative approach using a combination of ion exchange chromatography and gel filtration was also carried out to identify the bacterial myrosinase of E. -
Discovery of a Bacterial Glycoside Hydrolase Family 3 (GH3
Subscriber access provided by Imperial College London | Library Article Discovery of a bacterial glycoside hydrolase family 3 (GH3) #-glucosidase with myrosinase activity from a Citrobacter strain isolated from soil Abdulhadi Albaser, Eleanna Kazana, Mark Bennett, Fatma Cebeci, Vijitra Luang-In, Pietro D. Spanu, and John T. Rossiter J. Agric. Food Chem., Just Accepted Manuscript • DOI: 10.1021/acs.jafc.5b05381 • Publication Date (Web): 28 Jan 2016 Downloaded from http://pubs.acs.org on January 29, 2016 Just Accepted “Just Accepted” manuscripts have been peer-reviewed and accepted for publication. They are posted online prior to technical editing, formatting for publication and author proofing. The American Chemical Society provides “Just Accepted” as a free service to the research community to expedite the dissemination of scientific material as soon as possible after acceptance. “Just Accepted” manuscripts appear in full in PDF format accompanied by an HTML abstract. “Just Accepted” manuscripts have been fully peer reviewed, but should not be considered the official version of record. They are accessible to all readers and citable by the Digital Object Identifier (DOI®). “Just Accepted” is an optional service offered to authors. Therefore, the “Just Accepted” Web site may not include all articles that will be published in the journal. After a manuscript is technically edited and formatted, it will be removed from the “Just Accepted” Web site and published as an ASAP article. Note that technical editing may introduce minor changes to the manuscript text and/or graphics which could affect content, and all legal disclaimers and ethical guidelines that apply to the journal pertain. -
Disarming the Mustard Oil Bomb
Disarming the mustard oil bomb Andreas Ratzka*, Heiko Vogel*†, Daniel J. Kliebenstein‡, Thomas Mitchell-Olds*, and Juergen Kroymann* *Department of Genetics and Evolution, Max Planck Institute for Chemical Ecology, Winzerlaer Strasse 10, 07745 Jena, Germany; and ‡Department of Vegetable Crops, University of California, Davis, CA 95616 Edited by May R. Berenbaum, University of Illinois at Urbana–Champaign, Urbana, IL, and approved June 27, 2002 (received for review February 25, 2002) Plants are attacked by a broad array of herbivores and pathogens. In response, plants deploy an arsenal of defensive traits. In Bras- sicaceae, the glucosinolate–myrosinase complex is a sophisticated two-component system to ward off opponents. However, this so-called ‘‘mustard oil bomb’’ is disarmed by a glucosinolate sulfatase of a crucifer specialist insect, diamondback moth, Plutella xylostella (Lepidoptera: Plutellidae). Sulfatase activity of this en- zyme largely prevents the formation of toxic hydrolysis products arising from this plant defense system. Importantly, the enzyme acts on all major classes of glucosinolates, thus enabling diamond- back moths to use a broad range of cruciferous host plants. n response to biotic challenges, plants have evolved a broad Ivariety of defense mechanisms. These include preformed physical and chemical barriers, as well as inducible defenses. A well-studied example is the glucosinolate–myrosinase system (Fig. 1A), also referred to as ‘‘the mustard oil bomb’’ (1, 2). Cruciferous plants synthesize glucosinolates, a class of plant secondary compounds that share a core consisting of a -thio- Fig. 1. Reactions catalyzed by plant myrosinase and diamondback moth GSS. glucose moiety and a sulfonated oxime, but differ by a variable (A) Myrosinase removes glucose from glucosinolates (Top), leading to the side chain derived from one of several amino acids (3).