2021.03.31.437758V1.Full.Pdf
Total Page:16
File Type:pdf, Size:1020Kb
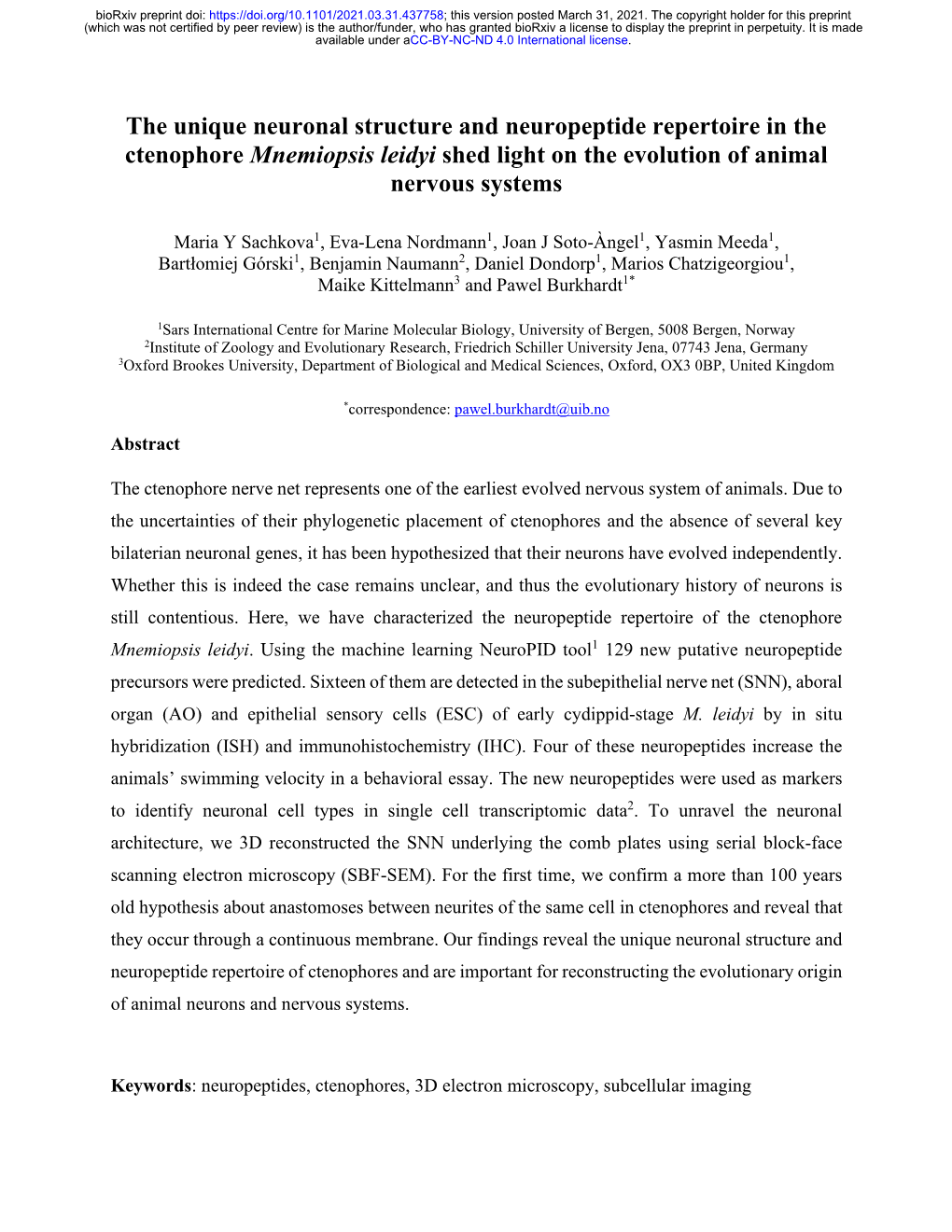
Load more
Recommended publications
-
Ctenophore Relationships and Their Placement As the Sister Group to All Other Animals
ARTICLES DOI: 10.1038/s41559-017-0331-3 Ctenophore relationships and their placement as the sister group to all other animals Nathan V. Whelan 1,2*, Kevin M. Kocot3, Tatiana P. Moroz4, Krishanu Mukherjee4, Peter Williams4, Gustav Paulay5, Leonid L. Moroz 4,6* and Kenneth M. Halanych 1* Ctenophora, comprising approximately 200 described species, is an important lineage for understanding metazoan evolution and is of great ecological and economic importance. Ctenophore diversity includes species with unique colloblasts used for prey capture, smooth and striated muscles, benthic and pelagic lifestyles, and locomotion with ciliated paddles or muscular propul- sion. However, the ancestral states of traits are debated and relationships among many lineages are unresolved. Here, using 27 newly sequenced ctenophore transcriptomes, publicly available data and methods to control systematic error, we establish the placement of Ctenophora as the sister group to all other animals and refine the phylogenetic relationships within ctenophores. Molecular clock analyses suggest modern ctenophore diversity originated approximately 350 million years ago ± 88 million years, conflicting with previous hypotheses, which suggest it originated approximately 65 million years ago. We recover Euplokamis dunlapae—a species with striated muscles—as the sister lineage to other sampled ctenophores. Ancestral state reconstruction shows that the most recent common ancestor of extant ctenophores was pelagic, possessed tentacles, was bio- luminescent and did not have separate sexes. Our results imply at least two transitions from a pelagic to benthic lifestyle within Ctenophora, suggesting that such transitions were more common in animal diversification than previously thought. tenophores, or comb jellies, have successfully colonized from species across most of the known phylogenetic diversity of nearly every marine environment and can be key species in Ctenophora. -
Role of Excess Inorganic Pyrophosphate in Primer-Extension Genotyping Assays
Methods Role of Excess Inorganic Pyrophosphate in Primer-Extension Genotyping Assays Ming Xiao,1 Angie Phong,1 Kristen L. Lum,1 Richard A. Greene,2 Philip R. Buzby,2,3 and Pui-Yan Kwok1,4,5 1Cardiovascular Research Institute, University of California, San Francisco, San Francisco, California 94143-0130, USA; 2PerkinElmer Life and Analytical Sciences, Inc., Boston, Massachusetts 02118-2512, USA We have developed and genotyped >15,000 SNP assays by using a primer extension genotyping assay with fluorescence polarization (FP) detection. Although the 80% success rate of this assay is similar to those of other SNP genotyping assays, we wanted to determine the reasons for the failures and find ways to improve the assay. We observed that the failed assays fell into three general patterns: PCR failure, excess of heterozygous genotypes, and loss of FP signal for one of the dye labels. After analyzing several hundred failed assays, we concluded that 5% of the assays had PCR failure and had to be redesigned. We also discovered that the other two categories of failures were due to misincorporation of one of the dye-terminators during the primer extension reaction as a result of primer shortening with a reverse reaction involving inorganic pyrophosphate, and to the quenching of R110-terminator after its incorporation onto the SNP primer. The relatively slow incorporation of R110 acycloterminators by AcycloPol compounds the problem with the R110 label. In this report, we describe the source of the problems and simple ways to correct these problems by adding pyrophosphatase, using quenching as part of the analysis, and replacing R110 by Texas red as one of the dye labels. -
Complexin Suppresses Spontaneous Exocytosis by Capturing the Membrane- Proximal Regions of VAMP2 and SNAP25
bioRxiv preprint doi: https://doi.org/10.1101/849885; this version posted November 21, 2019. The copyright holder for this preprint (which was not certified by peer review) is the author/funder. All rights reserved. No reuse allowed without permission. Complexin suppresses spontaneous exocytosis by capturing the membrane- proximal regions of VAMP2 and SNAP25 Authors: J. Malsam1,6, S. Bärfuss1,6, T. Trimbuch2, F. Zarebidaki2, A.F.-P. Sonnen3,5, K. Wild1, A. Scheutzow1, I. Sinning1, J.A.G. Briggs3,4, C. Rosenmund2, and T.H. Söllner1,7,* Author Affiliations: 1Heidelberg University Biochemistry Center, Im Neuenheimer Feld 328, 69120 Heidelberg, Germany. 2Neuroscience Research Center, Charité Universitätsmedizin Berlin, Chariteplatz 1, 10117 Berlin, Germany. 3European Molecular Biology Laboratory, Meyerhofstraße 1, 69117 Heidelberg, Germany. 4MRC Laboratory of Molecular Biology, Francis Crick Avenue, Cambridge Biomedical Campus, Cambridge CB2 0QH, UK. 5Present address: Department of Pathology, University Medical Centre Utrecht, Heidelberglaan 100, 3584 CX Utrecht, The Netherlands 6These authors contributed equally 7Lead Contact *Correspondence: [email protected]. Summary The neuronal protein complexin contains multiple domains that exert both clamping and facilitatory functions to tune spontaneous and action potential triggered synaptic release. We address the clamping mechanism and show that the accessory helix of complexin arrests the assembly of the soluble N-ethylmaleimide-sensitive factor attachment protein receptor -
Neuropeptidergic Signaling Partitions Arousal Behaviors in Zebrafish
3142 • The Journal of Neuroscience, February 26, 2014 • 34(9):3142–3160 Behavioral/Cognitive Neuropeptidergic Signaling Partitions Arousal Behaviors in Zebrafish Ian G. Woods,1,2 David Schoppik,2 Veronica J. Shi,2 Steven Zimmerman,2 Haley A. Coleman,1 Joel Greenwood,3 Edward R. Soucy,3 and Alexander F. Schier2,3 1Department of Biology, Ithaca College, Ithaca, New York 14850, and 2Department of Molecular and Cellular Biology and 3Center for Brain Science, Harvard University, Cambridge, Massachusetts 02138 Animals modulate their arousal state to ensure that their sensory responsiveness and locomotor activity match environmental demands. Neuropeptides can regulate arousal, but studies of their roles in vertebrates have been constrained by the vast array of neuropeptides and their pleiotropic effects. To overcome these limitations, we systematically dissected the neuropeptidergic modulation of arousal in larval zebrafish. We quantified spontaneous locomotor activity and responsiveness to sensory stimuli after genetically induced expression of seven evolutionarily conserved neuropeptides, including adenylate cyclase activating polypeptide 1b (adcyap1b), cocaine-related and amphetamine-related transcript (cart), cholecystokinin (cck), calcitonin gene-related peptide (cgrp), galanin, hypocretin, and nocicep- tin. Our study reveals that arousal behaviors are dissociable: neuropeptide expression uncoupled spontaneous activity from sensory responsiveness, and uncovered modality-specific effects upon sensory responsiveness. Principal components analysis and phenotypic clustering revealed both shared and divergent features of neuropeptidergic functions: hypocretin and cgrp stimulated spontaneous locomotor activity, whereas galanin and nociceptin attenuated these behaviors. In contrast, cart and adcyap1b enhanced sensory respon- siveness yet had minimal impacts on spontaneous activity, and cck expression induced the opposite effects. Furthermore, hypocretin and nociceptin induced modality-specific differences in responsiveness to changes in illumination. -
C-Terminal Domain of Mammalian Complexin-1 Localizes to Highly Curved Membranes
C-terminal domain of mammalian complexin-1 localizes to highly curved membranes Jihong Gonga,b,c,d,1, Ying Laie,1, Xiaohong Lia,b,c,d, Mengxian Wanga,b,c,d, Jeremy Leitze,f, Yachong Hug, Yunxiang Zhange,UcheorB.Choie, Daniel Ciprianoe,f, Richard A. Pfuetznere,f, Thomas C. Südhofe,f, Xiaofei Yanga,b,c,d,2, Axel T. Brungere,f,h,i,j,2, and Jiajie Diaoe,f,2,3 aKey Laboratory of Cognitive Science, College of Biomedical Engineering, South-Central University for Nationalities, Wuhan 430074, China; bHubei Key Laboratory of Medical Information Analysis and Tumor Diagnosis & Treatment, College of Biomedical Engineering, South-Central University for Nationalities, Wuhan 430074, China; cLaboratory of Membrane Ion Channels and Medicine, College of Biomedical Engineering, South-Central University for Nationalities, Wuhan 430074, China; dCollege of Life Science, South-Central University for Nationalities, Wuhan 430074, China; eDepartment of Molecular and Cellular Physiology, Stanford University, Stanford, CA 94305; fHoward Hughes Medical Institute, Stanford University, Stanford, CA 94305; gCenter for Mitochondrial Biology and Medicine, The Key Laboratory of Biomedical Information Engineering of Ministry of Education, School of Life Science and Technology, Xi’an Jiaotong University, Xi’an 710049, China; hDepartment of Neurology and Neurological Sciences, Stanford University, Stanford, CA 94305; iDepartment of Structural Biology, Stanford University, Stanford, CA 94305; and jDepartment of Photon Science, Stanford University, Stanford, CA 94305 Contributed by Axel T. Brunger, August 25, 2016 (sent for review June 21, 2016; reviewed by Jeremy S. Dittman and Erdem Karatekin) In presynaptic nerve terminals, complexin regulates spontaneous membranes via a membrane-binding motif (20, 21). -
Figure S1. HAEC ROS Production and ML090 NOX5-Inhibition
Figure S1. HAEC ROS production and ML090 NOX5-inhibition. (a) Extracellular H2O2 production in HAEC treated with ML090 at different concentrations and 24 h after being infected with GFP and NOX5-β adenoviruses (MOI 100). **p< 0.01, and ****p< 0.0001 vs control NOX5-β-infected cells (ML090, 0 nM). Results expressed as mean ± SEM. Fold increase vs GFP-infected cells with 0 nM of ML090. n= 6. (b) NOX5-β overexpression and DHE oxidation in HAEC. Representative images from three experiments are shown. Intracellular superoxide anion production of HAEC 24 h after infection with GFP and NOX5-β adenoviruses at different MOIs treated or not with ML090 (10 nM). MOI: Multiplicity of infection. Figure S2. Ontology analysis of HAEC infected with NOX5-β. Ontology analysis shows that the response to unfolded protein is the most relevant. Figure S3. UPR mRNA expression in heart of infarcted transgenic mice. n= 12-13. Results expressed as mean ± SEM. Table S1: Altered gene expression due to NOX5-β expression at 12 h (bold, highlighted in yellow). N12hvsG12h N18hvsG18h N24hvsG24h GeneName GeneDescription TranscriptID logFC p-value logFC p-value logFC p-value family with sequence similarity NM_052966 1.45 1.20E-17 2.44 3.27E-19 2.96 6.24E-21 FAM129A 129. member A DnaJ (Hsp40) homolog. NM_001130182 2.19 9.83E-20 2.94 2.90E-19 3.01 1.68E-19 DNAJA4 subfamily A. member 4 phorbol-12-myristate-13-acetate- NM_021127 0.93 1.84E-12 2.41 1.32E-17 2.69 1.43E-18 PMAIP1 induced protein 1 E2F7 E2F transcription factor 7 NM_203394 0.71 8.35E-11 2.20 2.21E-17 2.48 1.84E-18 DnaJ (Hsp40) homolog. -
TBC1D8B Mutations Implicate RAB11-Dependent Vesicular Trafficking in the Pathogenesis of Nephrotic Syndrome
BASIC RESEARCH www.jasn.org TBC1D8B Mutations Implicate RAB11-Dependent Vesicular Trafficking in the Pathogenesis of Nephrotic Syndrome Lina L. Kampf,1 Ronen Schneider,2 Lea Gerstner,1 Roland Thünauer,3,4 Mengmeng Chen,1 Martin Helmstädter,1 Ali Amar,2 Ana C. Onuchic-Whitford,2,5 Reyner Loza Munarriz,6 Afig Berdeli,7 Dominik Müller,8 Eva Schrezenmeier,9 Klemens Budde,9 Shrikant Mane,10 Kristen M. Laricchia,11 Heidi L. Rehm ,11 Daniel G. MacArthur,11 Richard P. Lifton,10,12 Gerd Walz,1 Winfried Römer,3 Carsten Bergmann,13,14,15 Friedhelm Hildebrandt,2 and Tobias Hermle 1 Due to the number of contributing authors, the affiliations are listed at the end of this article. ABSTRACT Background Mutations in about 50 genes have been identified as monogenic causes of nephrotic syndrome, a frequent cause of CKD. These genes delineated the pathogenetic pathways and rendered significant insight into podocyte biology. Methods We used whole-exome sequencing to identify novel monogenic causes of steroid-resistant nephrotic syndrome (SRNS). We analyzed the functional significance of an SRNS-associated gene in vitro and in podocyte-like Drosophila nephrocytes. Results We identified hemizygous missense mutations in the gene TBC1D8B in five families with nephrotic syndrome. Coimmunoprecipitation assays indicated interactions between TBC1D8B and active forms of RAB11. Silencing TBC1D8B in HEK293T cells increased basal autophagy and exocytosis, two cellular functions that are independently regulated by RAB11. This suggests that TBC1D8B plays a regulatory role by inhibiting endogenous RAB11. Coimmunoprecipitation assays showed TBC1D8B also interacts with the slit diaphragm protein nephrin, and colocalizes with it in immortalized cell lines. -
New Genomic Data and Analyses Challenge the Traditional Vision of Animal Epithelium Evolution
New genomic data and analyses challenge the traditional vision of animal epithelium evolution Hassiba Belahbib, Emmanuelle Renard, Sébastien Santini, Cyril Jourda, Jean-Michel Claverie, Carole Borchiellini, André Le Bivic To cite this version: Hassiba Belahbib, Emmanuelle Renard, Sébastien Santini, Cyril Jourda, Jean-Michel Claverie, et al.. New genomic data and analyses challenge the traditional vision of animal epithelium evolution. BMC Genomics, BioMed Central, 2018, 19 (1), pp.393. 10.1186/s12864-018-4715-9. hal-01951941 HAL Id: hal-01951941 https://hal-amu.archives-ouvertes.fr/hal-01951941 Submitted on 11 Dec 2018 HAL is a multi-disciplinary open access L’archive ouverte pluridisciplinaire HAL, est archive for the deposit and dissemination of sci- destinée au dépôt et à la diffusion de documents entific research documents, whether they are pub- scientifiques de niveau recherche, publiés ou non, lished or not. The documents may come from émanant des établissements d’enseignement et de teaching and research institutions in France or recherche français ou étrangers, des laboratoires abroad, or from public or private research centers. publics ou privés. Distributed under a Creative Commons Attribution| 4.0 International License Belahbib et al. BMC Genomics (2018) 19:393 https://doi.org/10.1186/s12864-018-4715-9 RESEARCHARTICLE Open Access New genomic data and analyses challenge the traditional vision of animal epithelium evolution Hassiba Belahbib1, Emmanuelle Renard2, Sébastien Santini1, Cyril Jourda1, Jean-Michel Claverie1*, Carole Borchiellini2* and André Le Bivic3* Abstract Background: The emergence of epithelia was the foundation of metazoan expansion. Epithelial tissues are a hallmark of metazoans deeply rooted in the evolution of their complex developmental morphogenesis processes. -
Title BOLINOPSIS RUBRIPUNCTATA N. SP., a NEW LOBATEAN
View metadata, citation and similar papers at core.ac.uk brought to you by CORE provided by Kyoto University Research Information Repository BOLINOPSIS RUBRIPUNCTATA N. SP., A NEW Title LOBATEAN CTENOPHORE FROM SETO Author(s) Tokioka, Takasi PUBLICATIONS OF THE SETO MARINE BIOLOGICAL Citation LABORATORY (1964), 12(1): 93-99 Issue Date 1964-06-30 URL http://hdl.handle.net/2433/175350 Right Type Departmental Bulletin Paper Textversion publisher Kyoto University BOLINOPSIS RUBRIPUNCTATA N. SP., A NEW LOBATEAN CTENOPHORE FROM SETO l) TAKAS! TOKIOKA Seto Marine Biological Laboratory With 3 Text-figures On the early afternoon of January 6 this year Mr. Torao YAMAMOTO brought me a perfect living Cyanea nozakii KrsHINOUYE of a medium-size caught inside the wharf at the fishing port of Seto about 1 km east of the laboratory and told me that many ctenophores were gathered near the northwest corner of the port together with some cyaneas. In a hope to get another good specimen of Cyanea or to find out some interesting medusae, I followed him to the port by bicycle and made a close observation there. The swarm there formed was composed mainly of Bolinopsis mikado (MosER), a significant number of Ocyropsis fusca RANG and some of Leucothea japonica KoMAr, Cestum amphitrites MERTENS and Beroe cucumis FABRICIUS, besides a considerable amount of Noctiluca and some hydromedusae. Among those ctenophores I found two specimens of a lobatean of a moderate size which were respectively marked distinctly with scarlet lines and large bright red spots up to about 15 in number. One of them was caught by bucket and the other by polyethylene bag, and then both specimens were carried to the laboratory on foot. -
An Annotated Checklist of the Marine Macroinvertebrates of Alaska David T
NOAA Professional Paper NMFS 19 An annotated checklist of the marine macroinvertebrates of Alaska David T. Drumm • Katherine P. Maslenikov Robert Van Syoc • James W. Orr • Robert R. Lauth Duane E. Stevenson • Theodore W. Pietsch November 2016 U.S. Department of Commerce NOAA Professional Penny Pritzker Secretary of Commerce National Oceanic Papers NMFS and Atmospheric Administration Kathryn D. Sullivan Scientific Editor* Administrator Richard Langton National Marine National Marine Fisheries Service Fisheries Service Northeast Fisheries Science Center Maine Field Station Eileen Sobeck 17 Godfrey Drive, Suite 1 Assistant Administrator Orono, Maine 04473 for Fisheries Associate Editor Kathryn Dennis National Marine Fisheries Service Office of Science and Technology Economics and Social Analysis Division 1845 Wasp Blvd., Bldg. 178 Honolulu, Hawaii 96818 Managing Editor Shelley Arenas National Marine Fisheries Service Scientific Publications Office 7600 Sand Point Way NE Seattle, Washington 98115 Editorial Committee Ann C. Matarese National Marine Fisheries Service James W. Orr National Marine Fisheries Service The NOAA Professional Paper NMFS (ISSN 1931-4590) series is pub- lished by the Scientific Publications Of- *Bruce Mundy (PIFSC) was Scientific Editor during the fice, National Marine Fisheries Service, scientific editing and preparation of this report. NOAA, 7600 Sand Point Way NE, Seattle, WA 98115. The Secretary of Commerce has The NOAA Professional Paper NMFS series carries peer-reviewed, lengthy original determined that the publication of research reports, taxonomic keys, species synopses, flora and fauna studies, and data- this series is necessary in the transac- intensive reports on investigations in fishery science, engineering, and economics. tion of the public business required by law of this Department. -
Biogeography of Jellyfish in the North Atlantic, by Traditional and Genomic Methods
Earth Syst. Sci. Data, 7, 173–191, 2015 www.earth-syst-sci-data.net/7/173/2015/ doi:10.5194/essd-7-173-2015 © Author(s) 2015. CC Attribution 3.0 License. Biogeography of jellyfish in the North Atlantic, by traditional and genomic methods P. Licandro1, M. Blackett1,2, A. Fischer1, A. Hosia3,4, J. Kennedy5, R. R. Kirby6, K. Raab7,8, R. Stern1, and P. Tranter1 1Sir Alister Hardy Foundation for Ocean Science (SAHFOS), The Laboratory, Citadel Hill, Plymouth PL1 2PB, UK 2School of Ocean and Earth Science, National Oceanography Centre, University of Southampton, European Way, Southampton SO14 3ZH, UK 3University Museum of Bergen, Department of Natural History, University of Bergen, P.O. Box 7800, 5020 Bergen, Norway 4Institute of Marine Research, P.O. Box 1870, 5817 Nordnes, Bergen, Norway 5Department of Environment, Fisheries and Sealing Division, Box 1000 Station 1390, Iqaluit, Nunavut, XOA OHO, Canada 6Marine Institute, Plymouth University, Drake Circus, Plymouth PL4 8AA, UK 7Institute for Marine Resources and Ecosystem Studies (IMARES), P.O. Box 68, 1970 AB Ijmuiden, the Netherlands 8Wageningen University and Research Centre, P.O. Box 9101, 6700 HB Wageningen, the Netherlands Correspondence to: P. Licandro ([email protected]) Received: 26 February 2014 – Published in Earth Syst. Sci. Data Discuss.: 5 November 2014 Revised: 30 April 2015 – Accepted: 14 May 2015 – Published: 15 July 2015 Abstract. Scientific debate on whether or not the recent increase in reports of jellyfish outbreaks represents a true rise in their abundance has outlined a lack of reliable records of Cnidaria and Ctenophora. Here we describe different jellyfish data sets produced within the EU programme EURO-BASIN. -
The Ctenophore Mnemiopsis Leidyi A. Agassiz 1865 in Coastal Waters of the Netherlands: an Unrecognized Invasion?
Aquatic Invasions (2006) Volume 1, Issue 4: 270-277 DOI 10.3391/ai.2006.1.4.10 © 2006 The Author(s) Journal compilation © 2006 REABIC (http://www.reabic.net) This is an Open Access article Research article The ctenophore Mnemiopsis leidyi A. Agassiz 1865 in coastal waters of the Netherlands: an unrecognized invasion? Marco A. Faasse1 and Keith M. Bayha2* 1National Museum of Natural History Naturalis, P.O.Box 9517, 2300 RA Leiden, The Netherlands E-mail: [email protected] 2Dauphin Island Sea Lab, 101 Bienville Blvd., Dauphin Island, AL, 36528, USA E-mail: [email protected] *Corresponding author Received 3 December 2006; accepted in revised form 11 December 2006 Abstract The introduction of the American ctenophore Mnemiopsis leidyi to the Black Sea was one of the most dramatic of all marine bioinvasions and, in combination with eutrophication and overfishing, resulted in a total reorganization of the pelagic food web and significant economic losses. Given the impacts this animal has exhibited in its invaded habitats, the spread of this ctenophore to additional regions has been a topic of much consternation. Here, we show the presence of this invader in estuaries along the Netherlands coast, based both on morphological observation and molecular evidence (nuclear internal transcribed spacer region 1 [ITS-1] sequence). Furthermore, we suggest the possibility that this ctenophore may have been present in Dutch waters for several years, having been misidentified as the morphologically similar Bolinopsis infundibulum. Given the level of shipping activity in nearby ports (e.g. Antwerp and Rotterdam), we find it likely that M. leidyi found its way to the Dutch coast in the ballast water of cargo ships, as is thought for Mnemiopsis in the Black and Caspian Seas.