Management Strategies for Cyanobacteria (Blue-Green Algae): a Guide for Water Utilities
Total Page:16
File Type:pdf, Size:1020Kb
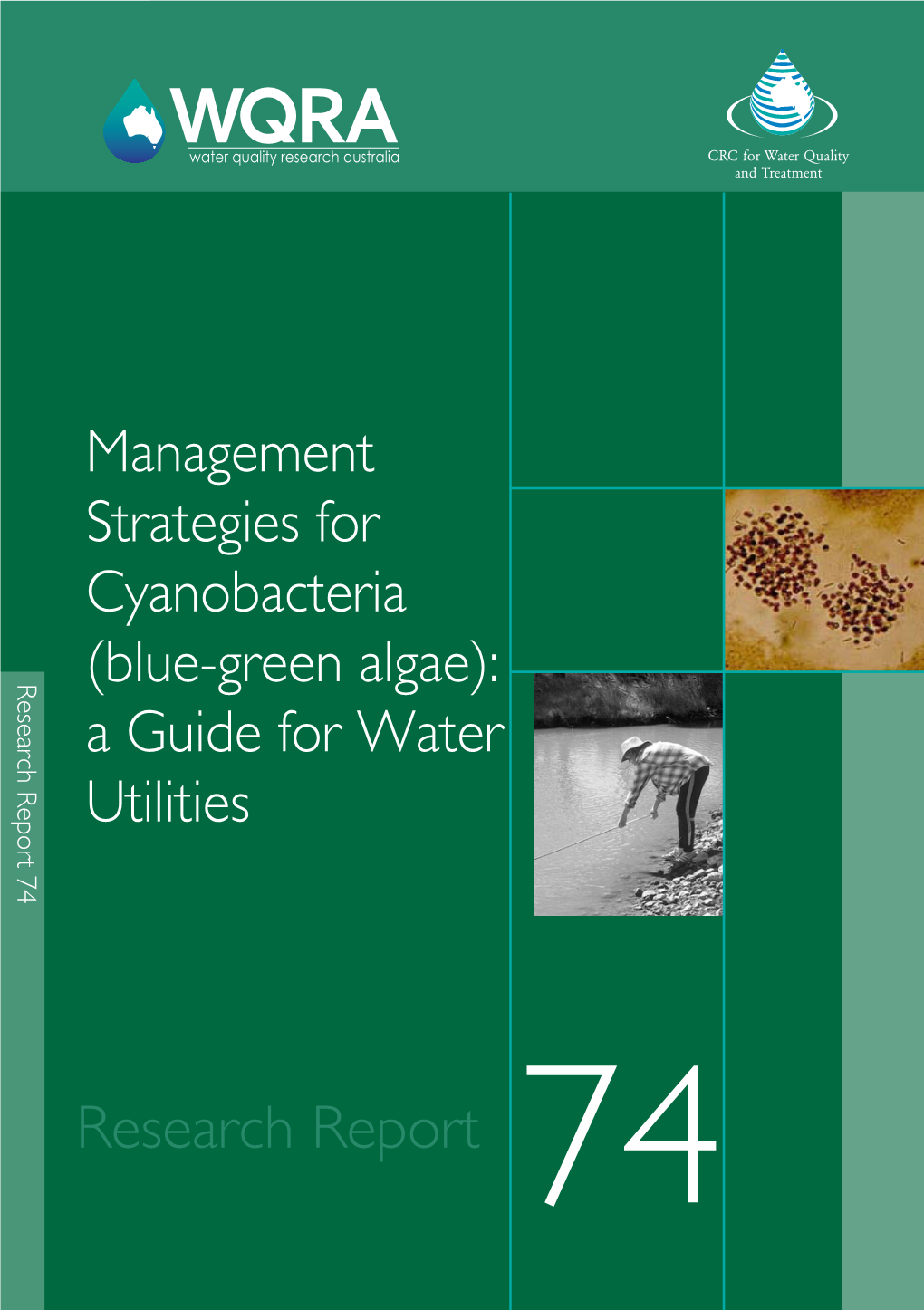
Load more
Recommended publications
-
The Influence of Probiotics on the Firmicutes/Bacteroidetes Ratio In
microorganisms Review The Influence of Probiotics on the Firmicutes/Bacteroidetes Ratio in the Treatment of Obesity and Inflammatory Bowel disease Spase Stojanov 1,2, Aleš Berlec 1,2 and Borut Štrukelj 1,2,* 1 Faculty of Pharmacy, University of Ljubljana, SI-1000 Ljubljana, Slovenia; [email protected] (S.S.); [email protected] (A.B.) 2 Department of Biotechnology, Jožef Stefan Institute, SI-1000 Ljubljana, Slovenia * Correspondence: borut.strukelj@ffa.uni-lj.si Received: 16 September 2020; Accepted: 31 October 2020; Published: 1 November 2020 Abstract: The two most important bacterial phyla in the gastrointestinal tract, Firmicutes and Bacteroidetes, have gained much attention in recent years. The Firmicutes/Bacteroidetes (F/B) ratio is widely accepted to have an important influence in maintaining normal intestinal homeostasis. Increased or decreased F/B ratio is regarded as dysbiosis, whereby the former is usually observed with obesity, and the latter with inflammatory bowel disease (IBD). Probiotics as live microorganisms can confer health benefits to the host when administered in adequate amounts. There is considerable evidence of their nutritional and immunosuppressive properties including reports that elucidate the association of probiotics with the F/B ratio, obesity, and IBD. Orally administered probiotics can contribute to the restoration of dysbiotic microbiota and to the prevention of obesity or IBD. However, as the effects of different probiotics on the F/B ratio differ, selecting the appropriate species or mixture is crucial. The most commonly tested probiotics for modifying the F/B ratio and treating obesity and IBD are from the genus Lactobacillus. In this paper, we review the effects of probiotics on the F/B ratio that lead to weight loss or immunosuppression. -
Guidelines for Design and Sampling for Cyanobacterial Toxin and Taste-And-Odor Studies in Lakes and Reservoirs
Guidelines for Design and Sampling for Cyanobacterial Toxin and Taste-and-Odor Studies in Lakes and Reservoirs Scientific Investigations Report 2008–5038 U.S. Department of the Interior U.S. Geological Survey Photo 1 Photo 3 Photo 2 Front cover. Photograph 1: Beach sign warning of the presence of a cyanobacterial bloom, June 29, 2006 (photograph taken by Jennifer L. Graham, U.S. Geological Survey). Photograph 2: Sampling a near-shore accumulation of Microcystis, August 8, 2006 (photograph taken by Jennifer L. Graham, U.S. Geological Survey). Photograph 3: Mixed bloom of Anabaena, Aphanizomenon, and Microcystis, August 10, 2006 (photograph taken by Jennifer L. Graham, U.S. Geological Survey). Background photograph: Near-shore accumulation of Microcystis, August 8, 2006 (photograph taken by Jennifer L. Graham, U.S. Geological Survey). Guidelines for Design and Sampling for Cyanobacterial Toxin and Taste-and-Odor Studies in Lakes and Reservoirs By Jennifer L. Graham, Keith A. Loftin, Andrew C. Ziegler, and Michael T. Meyer Scientific Investigations Report 2008–5038 U.S. Department of the Interior U.S. Geological Survey U.S. Department of the Interior DIRK KEMPTHORNE, Secretary U.S. Geological Survey Mark D. Myers, Director U.S. Geological Survey, Reston, Virginia: 2008 For product and ordering information: World Wide Web: http://www.usgs.gov/pubprod Telephone: 1-888-ASK-USGS For more information on the USGS—the Federal source for science about the Earth, its natural and living resources, natural hazards, and the environment: World Wide Web: http://www.usgs.gov Telephone: 1-888-ASK-USGS Any use of trade, product, or firm names is for descriptive purposes only and does not imply endorsement by the U.S. -
Cyanobacterial Toxins: Saxitoxins
WHO/SDE/WSH/xxxxx English only Cyanobacterial toxins: Saxitoxins Background document for development of WHO Guidelines for Drinking-water Quality and Guidelines for Safe Recreational Water Environments Version for Public Review Nov 2019 © World Health Organization 20XX Preface Information on cyanobacterial toxins, including saxitoxins, is comprehensively reviewed in a recent volume to be published by the World Health Organization, “Toxic Cyanobacteria in Water” (TCiW; Chorus & Welker, in press). This covers chemical properties of the toxins and information on the cyanobacteria producing them as well as guidance on assessing the risks of their occurrence, monitoring and management. In contrast, this background document focuses on reviewing the toxicological information available for guideline value derivation and the considerations for deriving the guideline values for saxitoxin in water. Sections 1-3 and 8 are largely summaries of respective chapters in TCiW and references to original studies can be found therein. To be written by WHO Secretariat Acknowledgements To be written by WHO Secretariat 5 Abbreviations used in text ARfD Acute Reference Dose bw body weight C Volume of drinking water assumed to be consumed daily by an adult GTX Gonyautoxin i.p. intraperitoneal i.v. intravenous LOAEL Lowest Observed Adverse Effect Level neoSTX Neosaxitoxin NOAEL No Observed Adverse Effect Level P Proportion of exposure assumed to be due to drinking water PSP Paralytic Shellfish Poisoning PST paralytic shellfish toxin STX saxitoxin STXOL saxitoxinol -
Yu-Chen Ling and John W. Moreau
Microbial Distribution and Activity in a Coastal Acid Sulfate Soil System Introduction: Bioremediation in Yu-Chen Ling and John W. Moreau coastal acid sulfate soil systems Method A Coastal acid sulfate soil (CASS) systems were School of Earth Sciences, University of Melbourne, Melbourne, VIC 3010, Australia formed when people drained the coastal area Microbial distribution controlled by environmental parameters Microbial activity showed two patterns exposing the soil to the air. Drainage makes iron Microbial structures can be grouped into three zones based on the highest similarity between samples (Fig. 4). Abundant populations, such as Deltaproteobacteria, kept constant activity across tidal cycling, whereas rare sulfides oxidize and release acidity to the These three zones were consistent with their geological background (Fig. 5). Zone 1: Organic horizon, had the populations changed activity response to environmental variations. Activity = cDNA/DNA environment, low pH pore water further dissolved lowest pH value. Zone 2: surface tidal zone, was influenced the most by tidal activity. Zone 3: Sulfuric zone, Abundant populations: the heavy metals. The acidity and toxic metals then Method A Deltaproteobacteria Deltaproteobacteria this area got neutralized the most. contaminate coastal and nearby ecosystems and Method B 1.5 cause environmental problems, such as fish kills, 1.5 decreased rice yields, release of greenhouse gases, Chloroflexi and construction damage. In Australia, there is Gammaproteobacteria Gammaproteobacteria about a $10 billion “legacy” from acid sulfate soils, Chloroflexi even though Australia is only occupied by around 1.0 1.0 Cyanobacteria,@ Acidobacteria Acidobacteria Alphaproteobacteria 18% of the global acid sulfate soils. Chloroplast Zetaproteobacteria Rare populations: Alphaproteobacteria Method A log(RNA(%)+1) Zetaproteobacteria log(RNA(%)+1) Method C Method B 0.5 0.5 Cyanobacteria,@ Bacteroidetes Chloroplast Firmicutes Firmicutes Bacteroidetes Planctomycetes Planctomycetes Ac8nobacteria Fig. -
Chapter 11 – PROKARYOTES: Survey of the Bacteria & Archaea
Chapter 11 – PROKARYOTES: Survey of the Bacteria & Archaea 1. The Bacteria 2. The Archaea Important Metabolic Terms Oxygen tolerance/usage: aerobic – requires or can use oxygen (O2) anaerobic – does not require or cannot tolerate O2 Energy usage: autotroph – uses CO2 as a carbon source • photoautotroph – uses light as an energy source • chemoautotroph – gets energy from inorganic mol. heterotroph – requires an organic carbon source • chemoheterotroph – gets energy & carbon from organic molecules …more Important Terms Facultative vs Obligate: facultative – “able to, but not requiring” e.g. • facultative anaerobes – can survive w/ or w/o O2 obligate – “absolutely requires” e.g. • obligate anaerobes – cannot tolerate O2 • obligate intracellular parasite – can only survive within a host cell The 2 Prokaryotic Domains Overview of the Bacterial Domain We will look at examples from several bacterial phyla grouped largely based on rRNA (ribotyping): Gram+ bacteria • Firmicutes (low G+C), Actinobacteria (high G+C) Proteobacteria (Gram- heterotrophs mainly) Gram- nonproteobacteria (photoautotrophs) Chlamydiae (no peptidoglycan in cell walls) Spirochaetes (coiled due to axial filaments) Bacteroides (mostly anaerobic) 1. The Gram+ Bacteria Gram+ Bacteria The Gram+ bacteria are found in 2 different phyla: Firmicutes • low G+C content (usually less than 50%) • many common pathogens Actinobacteria • high G+C content (greater than 50%) • characterized by branching filaments Firmicutes Characteristics associated with this phylum: • low G+C Gram+ bacteria -
Bacterial Avidins Are a Widely Distributed Protein Family in Actinobacteria, Proteobacteria and Bacteroidetes Olli H
Laitinen et al. BMC Ecol Evo (2021) 21:53 BMC Ecology and Evolution https://doi.org/10.1186/s12862-021-01784-y RESEARCH ARTICLE Open Access Bacterial avidins are a widely distributed protein family in Actinobacteria, Proteobacteria and Bacteroidetes Olli H. Laitinen1†, Tanja P. Kuusela1†, Sampo Kukkurainen1†, Anssi Nurminen1, Aki Sinkkonen2 and Vesa P. Hytönen1,3* Abstract Background: Avidins are biotin-binding proteins commonly found in the vertebrate eggs. In addition to streptavidin from Streptomyces avidinii, a growing number of avidins have been characterized from divergent bacterial species. However, a systematic research concerning their taxonomy and ecological role has never been done. We performed a search for avidin encoding genes among bacteria using available databases and classifed potential avidins according to taxonomy and the ecological niches utilized by host bacteria. Results: Numerous avidin-encoding genes were found in the phyla Actinobacteria and Proteobacteria. The diversity of protein sequences was high and several new variants of genes encoding biotin-binding avidins were found. The living strategies of bacteria hosting avidin encoding genes fall mainly into two categories. Human and animal patho- gens were overrepresented among the found bacteria carrying avidin genes. The other widespread category were bacteria that either fx nitrogen or live in root nodules/rhizospheres of plants hosting nitrogen-fxing bacteria. Conclusions: Bacterial avidins are a taxonomically and ecologically diverse group mainly found in Actinobacteria, Proteobacteria and Bacteroidetes, associated often with plant invasiveness. Avidin encoding genes in plasmids hint that avidins may be horizontally transferred. The current survey may be used as a basis in attempts to understand the ecological signifcance of biotin-binding capacity. -
Thermophilic Carboxydotrophs and Their Applications in Biotechnology Springerbriefs in Microbiology
SPRINGER BRIEFS IN MICROBIOLOGY EXTREMOPHILIC BACTERIA Sonia M. Tiquia-Arashiro Thermophilic Carboxydotrophs and their Applications in Biotechnology SpringerBriefs in Microbiology Extremophilic Bacteria Series editors Sonia M. Tiquia-Arashiro, Dearborn, MI, USA Melanie Mormile, Rolla, MO, USA More information about this series at http://www.springer.com/series/11917 Sonia M. Tiquia-Arashiro Thermophilic Carboxydotrophs and their Applications in Biotechnology 123 Sonia M. Tiquia-Arashiro Department of Natural Sciences University of Michigan Dearborn, MI USA ISSN 2191-5385 ISSN 2191-5393 (electronic) ISBN 978-3-319-11872-7 ISBN 978-3-319-11873-4 (eBook) DOI 10.1007/978-3-319-11873-4 Library of Congress Control Number: 2014951696 Springer Cham Heidelberg New York Dordrecht London © The Author(s) 2014 This work is subject to copyright. All rights are reserved by the Publisher, whether the whole or part of the material is concerned, specifically the rights of translation, reprinting, reuse of illustrations, recitation, broadcasting, reproduction on microfilms or in any other physical way, and transmission or information storage and retrieval, electronic adaptation, computer software, or by similar or dissimilar methodology now known or hereafter developed. Exempted from this legal reservation are brief excerpts in connection with reviews or scholarly analysis or material supplied specifically for the purpose of being entered and executed on a computer system, for exclusive use by the purchaser of the work. Duplication of this publication or parts thereof is permitted only under the provisions of the Copyright Law of the Publisher’s location, in its current version, and permission for use must always be obtained from Springer. -
The Spatial and Temporal Distribution and Environmental Drivers Of
THE SPATIAL AND TEMPORAL DISTRIBUTION AND POTENTIAL ENVIRONMENTAL DRIVERS OF SAXITOXIN IN NORTHWEST OHIO Callie A. Nauman A Thesis Submitted to the Graduate College of Bowling Green State University in partial fulfillment of the requirements for the degree of MASTER OF SCIENCE May 2020 Committee: Timothy Davis, Advisor George Bullerjahn Justin Chaffin © 2020 Callie A. Nauman All Rights Reserved iii ABSTRACT Timothy Davis, Advisor Cyanobacterial harmful algal blooms threaten freshwater quality and human health around the world. One specific threat is the ability of some cyanobacteria to produce multiple types of toxins, including a range of neurotoxins called saxitoxins. While it is not completely understood, the general consensus is environmental factors like phosphorus, nitrogen, and light availability, may be driving forces in saxitoxin production. Recent surveys have determined saxitoxin and potential saxitoxin producing cyanobacterial species in both lakes and rivers across the United States and Ohio. Research evaluating benthic cyanobacterial blooms determined benthic cyanobacteria as a source for saxitoxin production in systems, specifically rivers. Currently, little is known about when, where, why, or who is producing saxitoxin in Ohio, and even less is known about the role benthic cyanobacterial blooms play in Ohio waterways. With increased detections of saxitoxin, the saxitoxin biosynthesis gene sxtA, and saxitoxin producing species in both the Western Basin of Lake Erie and the lake’s major tributary the Maumee River, seasonal sampling was conducted to monitor saxitoxin in both systems. The sampling took place from late spring to early autumn of 2018 and 2019. Monitoring including bi-/weekly water column sampling in the Maumee River and Lake Erie and Nutrient Diffusing Substrate (NDS) Experiments, were completed to evaluate saxitoxin, sxtA, potential environmental drivers, and benthic production. -
Levels of Firmicutes, Actinobacteria Phyla and Lactobacillaceae
agriculture Article Levels of Firmicutes, Actinobacteria Phyla and Lactobacillaceae Family on the Skin Surface of Broiler Chickens (Ross 308) Depending on the Nutritional Supplement and the Housing Conditions Paulina Cholewi ´nska 1,* , Marta Michalak 2, Konrad Wojnarowski 1 , Szymon Skowera 1, Jakub Smoli ´nski 1 and Katarzyna Czyz˙ 1 1 Institute of Animal Breeding, Wroclaw University of Environmental and Life Sciences, 51-630 Wroclaw, Poland; [email protected] (K.W.); [email protected] (S.S.); [email protected] (J.S.); [email protected] (K.C.) 2 Department of Animal Nutrition and Feed Management, Wroclaw University of Environmental and Life Sciences, 51-630 Wroclaw, Poland; [email protected] * Correspondence: [email protected] Abstract: The microbiome of animals, both in the digestive tract and in the skin, plays an important role in protecting the host. The skin is one of the largest surface organs for animals; therefore, the destabilization of the microbiota on its surface can increase the risk of diseases that may adversely af- fect animals’ health and production rates, including poultry. The aim of this study was to evaluate the Citation: Cholewi´nska,P.; Michalak, effect of nutritional supplementation in the form of fermented rapeseed meal and housing conditions M.; Wojnarowski, K.; Skowera, S.; on the level of selected bacteria phyla (Firmicutes, Actinobacteria, and family Lactobacillaceae). The Smoli´nski,J.; Czyz,˙ K. Levels of study was performed on 30 specimens of broiler chickens (Ross 308), individually kept in metabolic Firmicutes, Actinobacteria Phyla and cages for 36 days. They were divided into 5 groups depending on the feed received. -
Harmful Algal Blooms (Habs) and Desalination: a Guide to Impacts, Monitoring, and Management
Manuals and Guides 78 Harmful Algal Blooms (HABs) and Desalination: A Guide to Impacts, Monitoring, and Management Edited by: Donald M. Anderson, Siobhan F.E. Boerlage, Mike B. Dixon UNESCO Manuals and Guides 78 Intergovernmental Oceanographic Commission Harmful Algal Blooms (HABs) and Desalination: A Guide to Impacts, Monitoring and Management Edited by: Donald M. Anderson* Biology Department, Woods Hole Oceanographic Institution Woods Hole, MA 02543 USA Siobhan F. E. Boerlage Boerlage Consulting Gold Coast, Queensland, Australia Mike B. Dixon MDD Consulting, Kensington Calgary, Alberta, Canada *Corresponding Author’s email: [email protected] UNESCO 2017 Bloom prevention and control 7 BLOOM PREVENTION AND CONTROL Clarissa R. Anderson1, Kevin G. Sellner2, and Donald M. Anderson3 1University of California, Santa Cruz, Santa Cruz, CA USA 2Chesapeake Research Consortium, Edgewater MD USA 3Woods Hole Oceanographic Institution, Woods Hole MA USA 7.1 Introduction ........................................................................................................................................... 205 7.2 Bloom prevention .................................................................................................................................. 207 7.2.1 Nutrient load reduction .................................................................................................................. 207 7.2.2 Nutrient load ................................................................................................................................. -
Cyanobacteria and Cyanotoxin Analysis and Testing Services [As of 3/15/2016]
Cyanobacteria/Cyanotoxin Testing Services List Developed by the NEIWPCC HAB Workgroup (Northeast state health and environmental agency staff). The New England Interstate Water Pollution Control Commission is a non-profit organization established through an act of Congress in 1947. NEIWPCC strives to: coordinate activities and forums that encourage cooperation among the states; educate the public about key environmental issues; support research projects, train environmental professionals, and provide overall leadership in the management and protection of water quality. Cyanobacteria and Cyanotoxin Analysis and Testing Services [As of 3/15/2016] Disclaimer: Individuals and/or companies listed below have been identified as performing services related to cyanobacteria and cyanotoxin analysis. This should not be considered an endorsement by NEIWPCC or any state agency of their qualifications. Public Water Systems considering contracting with any individual or company should verify that they have the adequate training and are competent to perform the work for which they are being considered. Prices should be viewed as guidelines of what to expect for each lab, rather than final quotes – many offer discounts, e.g., for bulk samples. Academy of Natural Sciences – Phycology Section Patrick Center for Environmental Research 1900 Benjamin Franklin Parkway Philadelphia, PA 19103 Tel: (215) 299-1080 Fax: (215) 299-1079 Email General: [email protected] Email Don Charles: [email protected] Email Frank Acker (primary soft-algae taxonomist): [email protected] Services: Identification of algae and algal measurements/biovolume, cell counts, chlorophyll Pricing: (Can give estimate based on sample, and separate the phytoplankton and periphyton in terms of how they are processed) Semiquantitative count (relative abundance, five-point scale, rare to abundant) – $150-200 Algal identification (cell count, biovolume) – $440-550 Chlorophyll (fluorometer) – Call for cost Diatom count – $300 Aquatic Services, Wayne Carmichael, Ph.D. -
This Article Was Published in an Elsevier Journal. the Attached Copy
This article was published in an Elsevier journal. The attached copy is furnished to the author for non-commercial research and education use, including for instruction at the author’s institution, sharing with colleagues and providing to institution administration. Other uses, including reproduction and distribution, or selling or licensing copies, or posting to personal, institutional or third party websites are prohibited. In most cases authors are permitted to post their version of the article (e.g. in Word or Tex form) to their personal website or institutional repository. Authors requiring further information regarding Elsevier’s archiving and manuscript policies are encouraged to visit: http://www.elsevier.com/copyright Author's personal copy Available online at www.sciencedirect.com Geochimica et Cosmochimica Acta 72 (2008) 1396–1414 www.elsevier.com/locate/gca Okenane, a biomarker for purple sulfur bacteria (Chromatiaceae), and other new carotenoid derivatives from the 1640 Ma Barney Creek Formation Jochen J. Brocks a,*, Philippe Schaeffer b a Research School of Earth Sciences and Centre for Macroevolution and Macroecology, The Australian National University, Canberra, ACT 0200, Australia b Laboratoire de Ge´ochimie Bio-organique, CNRS UMR 7177, Ecole Europe´enne de Chimie, Polyme`res et Mate´riaux, 25 rue Becquerel, 67200 Strasbourg, France Received 20 June 2007; accepted in revised form 12 December 2007; available online 23 December 2007 Abstract Carbonates of the 1640 million years (Ma) old Barney Creek Formation (BCF), McArthur Basin, Australia, contain more than 22 different C40 carotenoid derivatives including lycopane, c-carotane, b-carotane, chlorobactane, isorenieratane, b-iso- renieratane, renieratane, b-renierapurpurane, renierapurpurane and the monoaromatic carotenoid okenane.