Testing Strong-Field Gravity with Space-Based Detectors
Total Page:16
File Type:pdf, Size:1020Kb
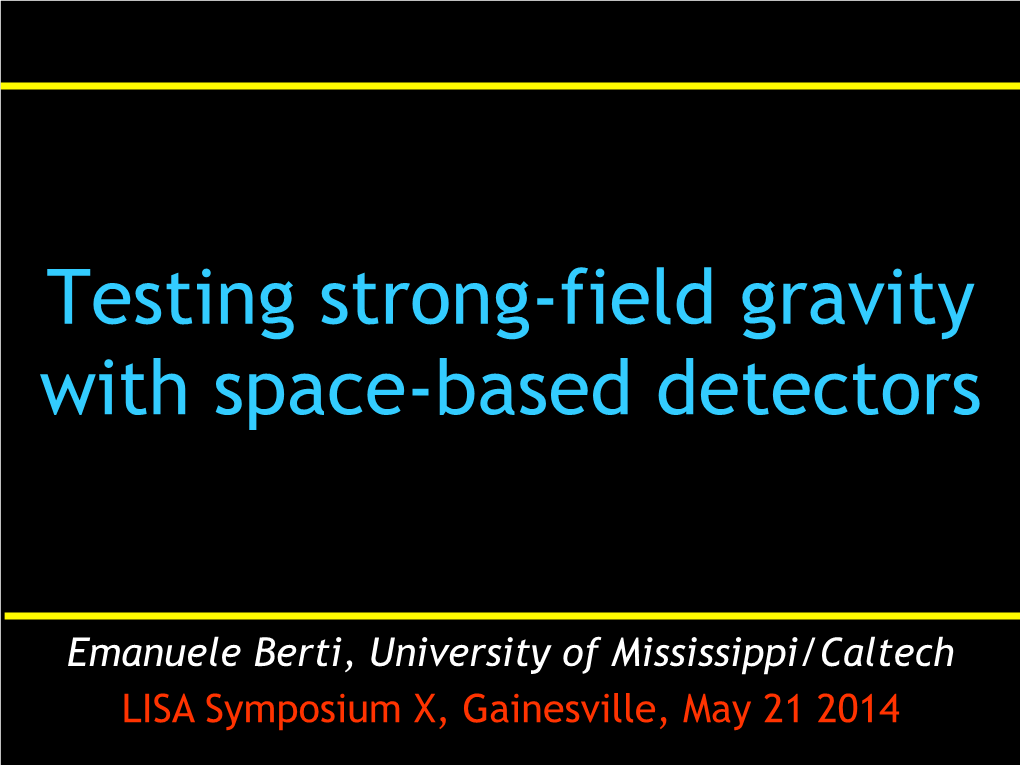
Load more
Recommended publications
-
Strong Field Dynamics of Bosonic Fields
Strong field dynamics of bosonic fields: Looking for new particles and modified gravity William East, Perimeter Institute ICERM Workshop October 26, 2020 ... Introduction How can we use gravitational waves to look for new matter? Can we come up with alternative predictions for black holes and/or GR to test against observations? Need understanding of relativistic/nonlinear dynamics for maximum return William East Strong field dynamics of bosonic fields ... Three examples with bosonic fields Black hole superradiance, boson stars, and modified gravity with non-minially coupled scalar fields William East Strong field dynamics of bosonic fields ... Gravitational wave probe of new particles Search new part of parameter space: ultralight particles weakly coupled to standard model William East Strong field dynamics of bosonic fields ... Superradiant instability: realizing the black hole bomb Massive bosons (scalar and vector) can form bound states, when frequency ! < mΩH grow exponentially in time. Search for new ultralight bosonic particles (axions, dark massive “photons," etc.) with Compton wavelength comparable to black hole radius (Arvanitaki et al.) William East Strong field dynamics of bosonic fields ... Boson clouds emit gravitational waves WE (2018) William East Strong field dynamics of bosonic fields ... Boson clouds emit gravitational waves 50 100 500 1000 5000 104 107 Can do targeted 105 1000 searches–e.g. follow-up 10 0.100 black hole merger events, 0.001 1 or “blind" searches 0.8 0.6 Look for either resolved or 0.4 1 stochastic sources with 0.98 0.96 LIGO (Baryakthar+ 2017; 0.94 Zhu+ 2020; Brito+ 2017; 10-12 10-11 10-10 Tsukada+ 2019) Siemonsen & WE (2020) William East Strong field dynamics of bosonic fields .. -
The Superradiant Stability of Kerr-Newman Black Holes
The superradiant stability of Kerr-Newman black holes Wen-Xiang Chen and Jing-Yi Zhang1∗ Department of Astronomy, School of Physics and Materials Science, GuangZhou University, Guangzhou 510006, China Yi-Xiao Zhang School of Information and Optoelectronic Science and Engineering, South China Normal University In this article, the superradiation stability of Kerr-Newman black holes is discussed by introducing the condition used in Kerr black holes y into them. Moreover, the motion equation of the minimal coupled scalar perturbation in a Kerr-Newman black hole is divided into angular and radial parts. We adopt the findings made by Erhart et al. on uncertainty principle in 2012, and discuss the bounds on y. 1. INTRODUCTION The No Hair Theorem of black holes first proposed in 1971 by Wheeler and proved by Stephen Hawking[1], Brandon Carter, etc, in 1973. In 1970s, the development of black hole thermodynamics applies the basic laws of thermodynamics to the theory of black hole in the field of general relativity, and strongly implies the profound and basic relationship among general relativity, thermodynamics and quantum theory. The stability of black holes is an major topic in black hole physics. Regge and Wheeler[2] have proved that the spherically symmetric Schwarzschild black hole is stable under perturbation. The great impact of superradiance makes the stability of rotating black holes more complicated. Superradiative effects occur in both classical and quantum scattering processes. When a boson wave hits a rotating black hole, chances are that rotating black holes are stable like Schwarzschild black holes, if certain conditions are satisfied[1–9] a ! < mΩH + qΦH ;ΩH = 2 2 (1) r+ + a where q and m are the charge and azimuthal quantum number of the incoming wave, ! denotes the wave frequency, ΩH is the angular velocity of black hole horizon and ΦH is the electromagnetic potential of the black hole horizon. -
The Extremal Black Hole Bomb
The extremal black hole bomb João G. Rosa Rudolf Peierls Centre for Theoretical Physics University of Oxford arXiv: 0912.1780 [hep‐th] "II Workshop on Black Holes", 22 December 2009, IST Lisbon. Introduction • Superradiant instability: Press & Teukolsky (1972-73) • scattering of waves in Kerr background (ergoregion) • f or modes with , scattered wave is amplified • Black hole bomb: Press & Teukolsky (1972), Cardoso et al. (2004), etc • multiple scatterings induced by mirror around BH • c an extract large amounts of energy and spin from the BH • Natural mirrors: • inner boundary of accretion disks Putten (1999), Aguirre (2000) • massive field bound states 22/12/09 The extremal black hole bomb, J. G. Rosa 2 Introduction • Estimates of superradiant instability growth rate (scalar field): • small mass limit: Detweiller (1980), Furuhashi & Nambu (2004) • lar ge mass limit: Zouros & Eardley (1979) • numeric al: Dolan (2007) 4 orders of magnitude • r ecent analytical: Hod & Hod (2009) discrepancy! 10 • µM 1 µ 10− eV Phenomenology: ∼ ⇒ ! • pions: mechanism effective if instability develops before decay, but only for small primordial black holes • “string axiverse”: Arvanitaki et al. (2009) many pseudomoduli acquire small masses from non‐perturbative string instantons and are much lighter than QCD axion! • Main goals: • c ompute superradiant spectrum of massive scalar states • f ocus on extremal BH and P‐wave modes (l=m=1) maximum growth rate • check discr epancy! 22/12/09 The extremal black hole bomb, J. G. Rosa 3 The massive Klein‐Gordon equation • Massive Klein‐Gordon equation in curved spacetime: • field decomposition: • angular part giv en in terms of spheroidal harmonics • Schrodinger‐like radial eq. -
String Theory, QCD and Black Holes
Black Hole Microstate Structure Fuzzballs and Firewalls Iosif Bena IPhT, CEAEA Saclay with Nick Warner, Jan deBoer, Micha Berkooz, Simon Ross, Gianguido Dall’Agata, Stefano Giusto, Masaki Shigemori, Dieter van den Bleeken, Monica Guica, Sheer El-Showk, Stanislav Kuperstein, Hagen Triendl, Bert Vercnocke, Andrea Puhm, Ref. Ares(2012)790792 - 29/06/2012 ! Department C "Grant Management" ! Mittwoch, 12. März 2014 ! Subject: Aggressive marketing from publishing houses Dear Madam, Dear Sir, The European Research Council Executive Agency (ERCEA) would like to alert you of publishing houses and online publications which try to make profit out of publishing articles, or interviewing researchers funded from the EU budget, either by the European Research Council (ERC) or by the European Commission (EC). From time to time participants in projects funded under the EU framework programmes are contacted – often by telephone - by organisations seeking payment in return for publishing information on the work being undertaken within their projects. As with "cold calling" in general, the claims and assertions made should be treated with an appropriate level of caution before a decision is made on the best course of action. These publications and their services have not been endorsed by the EC, or the ERCEA. Common tactics to secure business may include vague references to high-level contributions from decision makers, or making the project participant believe that his or her activities have been identified on the basis of special merit which may not be the case. Commercial entities use many ways of promoting their services, but grant-holders are not obliged to use them. Whatever the impression given by such service providers, their products have not received any formal approval or endorsement from the EC or the ERCEA. -
Schwarzschild Black Hole Can Also Produce Super-Radiation Phenomena
Schwarzschild black hole can also produce super-radiation phenomena Wen-Xiang Chen∗ Department of Astronomy, School of Physics and Materials Science, GuangZhou University According to traditional theory, the Schwarzschild black hole does not produce super radiation. If the boundary conditions are set in advance, the possibility is combined with the wave function of the coupling of the boson in the Schwarzschild black hole, and the mass of the incident boson acts as a mirror, so even if the Schwarzschild black hole can also produce super-radiation phenomena. Keywords: Schwarzschild black hole, superradiance, Wronskian determinant I. INTRODUCTION In a closely related study in 1962, Roger Penrose proposed a theory that by using point particles, it is possible to extract rotational energy from black holes. The Penrose process describes the fact that the Kerr black hole energy layer region may have negative energy relative to the observer outside the horizon. When a particle falls into the energy layer region, such a process may occur: the particle from the black hole To escape, its energy is greater than the initial state. It can also show that in Reissner-Nordstrom (charged, static) and rotating black holes, a generalized ergoregion and similar energy extraction process is possible. The Penrose process is a process inferred by Roger Penrose that can extract energy from a rotating black hole. Because the rotating energy is at the position of the black hole, not in the event horizon, but in the area called the energy layer in Kerr space-time, where the particles must be like a propelling locomotive, rotating with space-time, so it is possible to extract energy . -
The Weak Cosmic Censorship Conjecture May Be Violated
The weak cosmic censorship conjecture may be violated Wen-Xiang Chen∗ Department of Astronomy, School of Physics and Materials Science, GuangZhou University According to traditional theory, the Schwarzschild black hole does not produce superradiation. If the boundary conditions are set up in advance, this possibility will be combined with the boson- coupled wave function in the Schwarzschild black hole, where the incident boson will have a mirrored mass, so even the Schwarzschild black hole can generate superradiation phenomena.Recently, an article of mine obtained interesting results about the Schwarzschild black hole can generate super- radiation phenomena. The result contains some conclusions that violate the "no-hair theorem". We know that the phenomenon of black hole superradiation is a process of entropy reduction I found that the weak cosmic censorship conjecture may be violated. Keywords: weak cosmic censorship conjecture, entropy reduction, Schwarzschild-black-hole I. INTRODUCTION Since the physical behavior of the singularity is unknown, if the singularity can be observed from other time and space, the causality may be broken and physics may lose its predictive ability. This problem is unavoidable, because according to the Penrose-Hawking singularity theorem, singularities are unavoidable when physically reasonable. There is no naked singularity, the universe, as described by general relativity, is certain: it can predict the evolution of the entire universe (may not include some singularities hidden in the horizon of limited regional space), only knowing its state at a certain moment Time (more precisely, spacelike three-dimensional hypersurfaces are everywhere, called Cauchy surface). The failure of the cosmic censorship hypothesis leads to the failure of determinism, because it is still impossible to predict the causal future behavior of time and space at singularities. -
Arxiv:1605.04629V2 [Gr-Qc]
Implementing black hole as efficient power plant Shao-Wen Wei ∗, Yu-Xiao Liu† Institute of Theoretical Physics & Research Center of Gravitation, Lanzhou University, Lanzhou 730000, People’s Republic of China Abstract Treating the black hole molecules as working substance and considering its phase structure, we study the black hole heat engine by a charged anti-de Sitter black hole. In the reduced temperature- entropy chart, it is found that the work, heat, and efficiency of the engine are free of the black hole charge. Applying the Rankine cycle with or without a back pressure mechanism to the black hole heat engine, the compact formula for the efficiency is obtained. And the heat, work and efficiency are worked out. The result shows that the black hole engine working along the Rankine cycle with a back pressure mechanism has a higher efficiency. This provides a novel and efficient mechanism to produce the useful mechanical work, and such black hole heat engine may act as a possible energy source for the high energy astrophysical phenomena near the black hole. PACS numbers: 04.70.Dy, 05.70.Ce, 07.20.Pe Keywords: Black hole, heat engine, phase transition arXiv:1605.04629v2 [gr-qc] 7 Jun 2019 ∗ [email protected] † [email protected] 1 I. INTRODUCTION Black hole has been a fascinating object since general relativity predicted its existence. Compared with other stars, a black hole has sufficient density and a huge amount of energy. Near a black hole, there are many high energy astrophysical phenomena related to the energy flow, such as the power jet and black holes merging. -
Exotic Compact Objects Interacting with Fundamental Fields Engineering
Exotic compact objects interacting with fundamental fields Nuno André Moreira Santos Thesis to obtain the Master of Science Degree in Engineering Physics Supervisors: Prof. Dr. Carlos Alberto Ruivo Herdeiro Prof. Dr. Vítor Manuel dos Santos Cardoso Examination Committee Chairperson: Prof. Dr. José Pizarro de Sande e Lemos Supervisor: Prof. Dr. Carlos Alberto Ruivo Herdeiro Member of the Committee: Dr. Miguel Rodrigues Zilhão Nogueira October 2018 Resumo A astronomia de ondas gravitacionais apresenta-se como uma nova forma de testar os fundamentos da física − e, em particular, a gravidade. Os detetores de ondas gravitacionais por interferometria laser permitirão compreender melhor ou até esclarecer questões de longa data que continuam por responder, como seja a existência de buracos negros. Pese embora o número cumulativo de argumentos teóricos e evidências observacionais que tem vindo a fortalecer a hipótese da sua existência, não há ainda qualquer prova conclusiva. Os dados atualmente disponíveis não descartam a possibilidade de outros objetos exóticos, que não buracos negros, se formarem em resultado do colapso gravitacional de uma estrela suficientemente massiva. De facto, acredita-se que a assinatura do objeto exótico remanescente da coalescência de um sistema binário de objetos compactos pode estar encriptada na amplitude da onda gravitacional emitida durante a fase de oscilações amortecidas, o que tornaria possível a distinção entre buracos negros e outros objetos exóticos. Esta dissertação explora aspetos clássicos da fenomenologia de perturbações escalares e eletromagnéticas de duas famílias de objetos exóticos cuja geometria, apesar de semelhante à de um buraco negro de Kerr, é definida por uma superfície refletora, e não por um horizonte de eventos. -
Thesis by Saul Arno Teukolsky in Partial Fulfillment for the Degree Of
PERTURBATIONS OF A ROTATING BLACK HOLE Thesis by Saul Arno Teukolsky In Partial Fulfillment of the Requirements for the Degree of Doctor of Philosophy California Institute of Technology Pasadena, California 1974 (Submitted July 19, 1973) -ii- ACKNOWLEDGMENTS I thank my advisor, Kip Thorne, for his continued help and encouragement during the course of this work. Much of my progress was due to his example and teaching. I am especially in debted to Bill Press, who collaborated with me on four papers. I thank him not only for his collaboration, but also for innumerable discussions and helpful suggestions. I thank dim Bardeen for a number of useful discussions, and for his collaboration on a paper. For advice, help and discussions at various times, I wish to thank also Brandon Carter, S. Chandrasekhar, Paul Chrzanowski, Doug Eardley, George Fox, Jim Hartle, Steven Hawking, Jim Ipser, Herbert Keller, Sandor Kovacs, David Lee, Alan Lightman, Bonnie Miller, Charles Misner, Richard Price, Larry Smarr and Barbara Zimmerman. To my wife Roselyn go my thanks for moral support and encouragement at all times. I am grateful to the Pretoria Portland Cement Company for a scholarship during 1970 and to the United States Steel Foundation for a fellowship during 1972-73. Part of this research was sup ported by the National Science Foundation [GP-28027, GP-27304]. -πι- ABSTRACT Decoupled, separable equations describing perturbations of a Kerr black hole are derived. These equations can be used to study black-hole processes involving scalar, electromagnetic, neutrino or gravitational fields. A number of astrophysical applications are made: Misner's idea that gravitational synchro tron radiation might explain Weber's observations is shown to be untenable; rotating black holes are shown to be stable against small perturbations; energy amplification by "superradiant scat tering" of waves off a rotating black hole is computed; the "spin down" (loss of angular momentum) of a rotating black hole caused by a stationary non-axisymmetric perturbation is calculated. -
SNOWMASS21-TF1 TF0-057.Pdf 409.50KB 2020-08-31 21:46:42
Snowmass2021 - Letter of Interest Peering inside Black Holes with Gravitational Waves Thematic Areas: (TF01) String theory, quantum gravity, black holes (TF2) Effective field theory techniques (TF3) CFT and formal QFT (TF4) Scattering amplitudes (TF5) Lattice gauge theory (TF6) Theory techniques for precision physics (TF7) Collider phenomenology (TF8) BSM model building (TF9) Astro-particle physics & cosmology (TF10) Quantum Information Science (TF11) Theory of neutrino physics (CF6) Dark Energy and Cosmic Acceleration: Complementarity of Probes and New Facilities (CF7) Cosmic Probes of Fundamental Physics Contact Information: Emil Mottola (Los Alamos National Laboratory & Perimeter Institute)[[email protected]] Author: Emil Mottola Abstract: In classical General Relativity (GR) the interiors of Black Holes (BHs) are not only singular but, if rotating, also admit closed timelike curves, violating causality.1 This feature occurs at macroscopic distance scales, far larger than the microscopic Planck scale LP l. When quantum effects are considered, severe conflicts with statistical thermodynamics, conservation of probability and an enormous BH entropy arise also at the macroscopic horizon scale.2,3 This suggests that a low energy semi-classical Effective Field Theory (EFT) approach should be applicable. In this LOI such an approach based the conformal anomaly is proposed, which leads to a non-singular horizonless, but ultra-compact object called a gravitational condensate star. The gravastar hypothesis can be tested by searching for discrete surface modes and GW echoes emitted after binary merger events. In this new era of GW and multi-messenger astronomy with additional GW detectors coming online in this decade, the time is now ripe for a full-fledged effort to confront these theoretical ideas with the observational data that hold the promise of resolving the conundrum that BHs pose, and potentially point to a new path to ultimate synthesis of gravitation and quantum theory. -
Chronology Protection Conjecture May Not Hold Abstract
Chronology protection conjecture may not hold Wen-Xiang Chen ∗ Institute of quantum matter, School of Physics and Telecommunication Engineering, South China Normal University, Guangzhou 510006,China Abstract The cosmic censorship hypothesis has not been directly verified, and some physicists also question the validity of the cosmological censorship hypothesis. Through theoretical prediction, it pointed out that the existence of naked singularities is possible.This article puts forward a point that, under the quantum gravity equation, although there is no concept of wormholes, the chronology protection conjecture may not be true. Keywords: Chronology protection conjecture, entropy reduction, naked singularity I. INTRODUCTION The Chronology protection conjecture is the first hypothesis proposed by Stephen Hawking that the laws of physics can prevent the propagation of time on a microscopic scale. The admissibility of time travel is mathematically represented by the existence of closed time-like curves in certain solutions of the general relativistic field equations. The time protection conjecture should be different from the time check. Under the time check, each closed time-like curve passes the event range, which may prevent the observer from discovering causal conflicts (also known as time violations). As we all know, in general relativity, in principle, space-time is a possible space-time travel, that is, some trajectories will form a cycle with time, and the observers who follow them can return to their past. These cycles are called closed space-time curves (CTC). There is a close relationship between time travel and the speed at which the speed of light (super speed of light) in vacuum is greater. -
Publications of William H. Press 1. W.H. Press and J.M. Bardeen, “Nonconservation of the Newman-Penrose Conserved Quantities," Phys
Publications of William H. Press 1. W.H. Press and J.M. Bardeen, “Nonconservation of the Newman-Penrose Conserved Quantities," Phys. Rev. Lett., 27, 1303 (1971). 2. M. Davis, R. Ruffini, W. Press and R. Price, “Gravitational Radiation from a Particle Falling Radially into a Schwarzschild Black Hole," Phys. Rev. Lett., 27,1466 (1971). 3. W.H. Press, “Long Wave-Trains of Gravitational Waves from a Vibrating Black Hole," Astrophysics. J. (Lett.), 170, L105 (1971). 4. W.H. Press, “Time Evolution of a Rotating Black Hole Immersed in a Static Scalar Field," Astrophys. J., 175, 243 (1972). 5. W.H. Press and K.S. Thorne, “Gravitational-Wave Astronomy," Ann. Rev. Astron. Astrophys., 10, 335 (1972). 6. J.M. Bardeen, W.H. Press, and S.A. Teukolsky, “Rotating Black Holes: Locally Non- rotating Frames, Energy Extraction, and Scalar Synchrotron Radiation," Astrophys. J., 178, 347 (1972). 7. W.H. Press and S.A. Teukolsky, “Floating Orbits, Superradiant Scattering and the Black-Hole Bomb," Nature, 238, 211 (1972). 8. J.M. Bardeen and W.H. Press, “Radiation Fields in the Schwarzschild Background," J. Math. Phys., 14, 7 (1973). 9. W.H. Press and S.A. Teukolsky, “On the Evolution of the Secularly Unstable, Viscous Maclaurin Spheroids," Astrophys. J., 181, 513 (1973). 10. W.H. Press, “Black-Hole Perturbations: An Overview," in Proceedings of the Sixth Texas Symposium on Relativistic Astrophysics, Ann. N.Y. Acad. Sci., 224, 272 (1973). 11. W.H.Press and S.A. Teukolsky, “Perturbations of a Rotating Black Hole. II. Dynamical Stability of the Kerr Metric," Astrophys. J. 185, 649 (1973).