The Pluto-New Horizons RTG and Power System Early Mission Performance
Total Page:16
File Type:pdf, Size:1020Kb
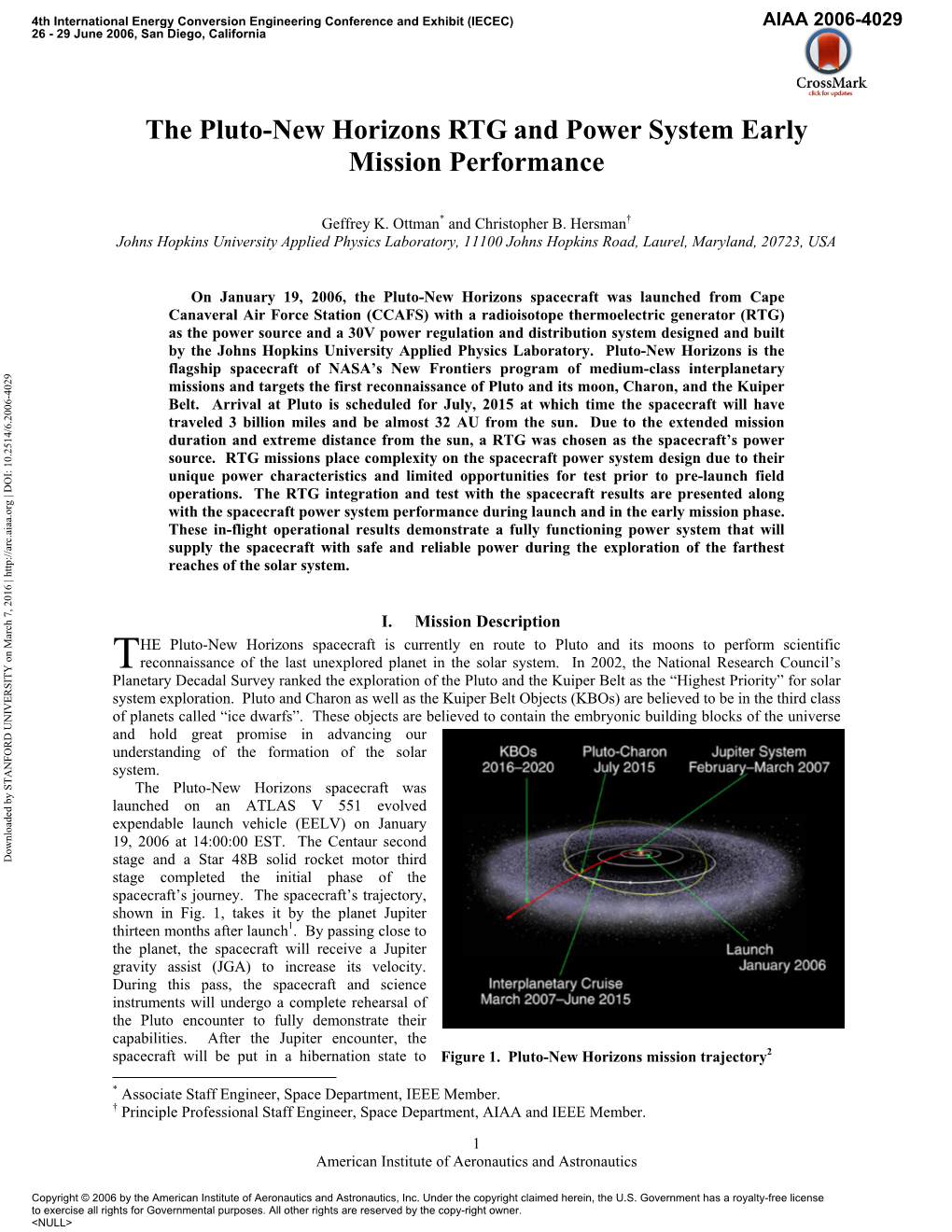
Load more
Recommended publications
-
New Horizons: Reconnaissance of the Pluto-Charon System and The
C.T. Russell Editor New Horizons Reconnaissance of the Pluto-Charon System and the Kuiper Belt Previously published in Space Science Reviews Volume 140, Issues 1–4, 2008 C.T. Russell Institute of Geophysics & Planetary Physics University of California Los Angeles, CA, USA Cover illustration: NASA’s New Horizons spacecraft was launched on 2006 January 19, received a grav- ity assist during a close approach to Jupiter on 2007 February 28, and is now headed for a flyby with closest approach 12,500 km from the center of Pluto on 2015 July 14. This artist’s depiction shows the spacecraft shortly after passing above Pluto’s highly variegated surface, which may have black-streaked surface deposits produced from cryogenic geyser activity, and just before passing into Pluto’s shadow when solar and earth occultation experiments will probe Pluto’s tenuous, and possibly hazy, atmosphere. Sunlit crescents of Pluto’s moons Charon, Nix, and Hydra are visible in the background. After flying through the Pluto system, the New Horizons spacecraft could be re-targeted towards other Kuiper Belt Objects in an extended mission phase. This image is based on an original painting by Dan Durda. © Dan Durda 2001 All rights reserved. Back cover illustration: The New Horizons spacecraft was launched aboard an Atlas 551 rocket from the NASA Kennedy Space Center on 2008 January 19 at 19:00 UT. Library of Congress Control Number: 2008944238 DOI: 10.1007/978-0-387-89518-5 ISBN-978-0-387-89517-8 e-ISBN-978-0-387-89518-5 Printed on acid-free paper. -
+ New Horizons
Media Contacts NASA Headquarters Policy/Program Management Dwayne Brown New Horizons Nuclear Safety (202) 358-1726 [email protected] The Johns Hopkins University Mission Management Applied Physics Laboratory Spacecraft Operations Michael Buckley (240) 228-7536 or (443) 778-7536 [email protected] Southwest Research Institute Principal Investigator Institution Maria Martinez (210) 522-3305 [email protected] NASA Kennedy Space Center Launch Operations George Diller (321) 867-2468 [email protected] Lockheed Martin Space Systems Launch Vehicle Julie Andrews (321) 853-1567 [email protected] International Launch Services Launch Vehicle Fran Slimmer (571) 633-7462 [email protected] NEW HORIZONS Table of Contents Media Services Information ................................................................................................ 2 Quick Facts .............................................................................................................................. 3 Pluto at a Glance ...................................................................................................................... 5 Why Pluto and the Kuiper Belt? The Science of New Horizons ............................... 7 NASA’s New Frontiers Program ........................................................................................14 The Spacecraft ........................................................................................................................15 Science Payload ...............................................................................................................16 -
1 the Atmosphere of Pluto As Observed by New Horizons G
The Atmosphere of Pluto as Observed by New Horizons G. Randall Gladstone,1,2* S. Alan Stern,3 Kimberly Ennico,4 Catherine B. Olkin,3 Harold A. Weaver,5 Leslie A. Young,3 Michael E. Summers,6 Darrell F. Strobel,7 David P. Hinson,8 Joshua A. Kammer,3 Alex H. Parker,3 Andrew J. Steffl,3 Ivan R. Linscott,9 Joel Wm. Parker,3 Andrew F. Cheng,5 David C. Slater,1† Maarten H. Versteeg,1 Thomas K. Greathouse,1 Kurt D. Retherford,1,2 Henry Throop,7 Nathaniel J. Cunningham,10 William W. Woods,9 Kelsi N. Singer,3 Constantine C. C. Tsang,3 Rebecca Schindhelm,3 Carey M. Lisse,5 Michael L. Wong,11 Yuk L. Yung,11 Xun Zhu,5 Werner Curdt,12 Panayotis Lavvas,13 Eliot F. Young,3 G. Leonard Tyler,9 and the New Horizons Science Team 1Southwest Research Institute, San Antonio, TX 78238, USA 2University of Texas at San Antonio, San Antonio, TX 78249, USA 3Southwest Research Institute, Boulder, CO 80302, USA 4National Aeronautics and Space Administration, Ames Research Center, Space Science Division, Moffett Field, CA 94035, USA 5The Johns Hopkins University Applied Physics Laboratory, Laurel, MD 20723, USA 6George Mason University, Fairfax, VA 22030, USA 7The Johns Hopkins University, Baltimore, MD 21218, USA 8Search for Extraterrestrial Intelligence Institute, Mountain View, CA 94043, USA 9Stanford University, Stanford, CA 94305, USA 10Nebraska Wesleyan University, Lincoln, NE 68504 11California Institute of Technology, Pasadena, CA 91125, USA 12Max-Planck-Institut für Sonnensystemforschung, 37191 Katlenburg-Lindau, Germany 13Groupe de Spectroscopie Moléculaire et Atmosphérique, Université Reims Champagne-Ardenne, 51687 Reims, France *To whom correspondence should be addressed. -
Anticipated Scientific Investigations at the Pluto System
Space Sci Rev (2008) 140: 93–127 DOI 10.1007/s11214-008-9462-9 New Horizons: Anticipated Scientific Investigations at the Pluto System Leslie A. Young · S. Alan Stern · Harold A. Weaver · Fran Bagenal · Richard P. Binzel · Bonnie Buratti · Andrew F. Cheng · Dale Cruikshank · G. Randall Gladstone · William M. Grundy · David P. Hinson · Mihaly Horanyi · Donald E. Jennings · Ivan R. Linscott · David J. McComas · William B. McKinnon · Ralph McNutt · Jeffery M. Moore · Scott Murchie · Catherine B. Olkin · Carolyn C. Porco · Harold Reitsema · Dennis C. Reuter · John R. Spencer · David C. Slater · Darrell Strobel · Michael E. Summers · G. Leonard Tyler Received: 5 January 2007 / Accepted: 28 October 2008 / Published online: 3 December 2008 © Springer Science+Business Media B.V. 2008 L.A. Young () · S.A. Stern · C.B. Olkin · J.R. Spencer Southwest Research Institute, Boulder, CO, USA e-mail: [email protected] H.A. Weaver · A.F. Cheng · R. McNutt · S. Murchie Johns Hopkins University Applied Physics Lab., Laurel, MD, USA F. Bagenal · M. Horanyi University of Colorado, Boulder, CO, USA R.P. Binzel Massachusetts Institute of Technology, Cambridge, MA, USA B. Buratti Jet Propulsion Laboratory, Pasadena, CA, USA D. Cruikshank · J.M. Moore NASA Ames Research Center, Moffett Field, CA, USA G.R. Gladstone · D.J. McComas · D.C. Slater Southwest Research Institute, San Antonio, TX, USA W.M. Grundy Lowell Observatory, Flagstaff, AZ, USA D.P. Hinson · I.R. Linscott · G.L. Tyler Stanford University, Stanford, CA, USA D.E. Jennings · D.C. Reuter NASA Goddard Space Flight Center, Greenbelt, MD, USA 94 L.A. Young et al. -
2018: Aiaa-Space-Report
AIAA TEAM SPACE TRANSPORTATION DESIGN COMPETITION TEAM PERSEPHONE Submitted By: Chelsea Dalton Ashley Miller Ryan Decker Sahil Pathan Layne Droppers Joshua Prentice Zach Harmon Andrew Townsend Nicholas Malone Nicholas Wijaya Iowa State University Department of Aerospace Engineering May 10, 2018 TEAM PERSEPHONE Page I Iowa State University: Persephone Design Team Chelsea Dalton Ryan Decker Layne Droppers Zachary Harmon Trajectory & Propulsion Communications & Power Team Lead Thermal Systems AIAA ID #908154 AIAA ID #906791 AIAA ID #532184 AIAA ID #921129 Nicholas Malone Ashley Miller Sahil Pathan Joshua Prentice Orbit Design Science Science Science AIAA ID #921128 AIAA ID #922108 AIAA ID #761247 AIAA ID #922104 Andrew Townsend Nicholas Wijaya Structures & CAD Trajectory & Propulsion AIAA ID #820259 AIAA ID #644893 TEAM PERSEPHONE Page II Contents 1 Introduction & Problem Background2 1.1 Motivation & Background......................................2 1.2 Mission Definition..........................................3 2 Mission Overview 5 2.1 Trade Study Tools..........................................5 2.2 Mission Architecture.........................................6 2.3 Planetary Protection.........................................6 3 Science 8 3.1 Observations of Interest.......................................8 3.2 Goals.................................................9 3.3 Instrumentation............................................ 10 3.3.1 Visible and Infrared Imaging|Ralph............................ 11 3.3.2 Radio Science Subsystem................................. -
DOE Could Improve Planning and Communication Related to Plutonium-238 And
United States Government Accountability Office Report to Congressional Requesters September 2017 SPACE EXPLORATION DOE Could Improve Planning and Communication Related to Plutonium- 238 and Radioisotope Power Systems Production Challenges Accessible Version GAO-17-673 September 2017 SPACE EXPLORATION DOE Could Improve Planning and Communication Related to Plutonium-238 and Radioisotope Power Systems Production Challenges Highlights of GAO-17-673, a report to congressional requesters Why GAO Did This Study What GAO Found NASA uses RPS to generate electrical The National Aeronautics and Space Administration (NASA) selects radioisotope power in missions in which solar power systems (RPS) for missions primarily based on the agency’s scientific panels or batteries would be objectives and mission destinations. Prior to the establishment of the Department ineffective. RPS convert heat of Energy’s (DOE) Supply Project in fiscal year 2011 to produce new plutonium- generated by the radioactive decay of 238 (Pu-238), NASA officials said that Pu-238 supply was a limiting factor in Pu-238 into electricity. DOE maintains selecting RPS-powered missions. After the initiation of the Supply Project, a capability to produce RPS for NASA however, NASA officials GAO interviewed said that missions are selected missions, as well as a limited and independently of decisions on how to power them. Once a mission is selected, aging supply of Pu-238 that will be NASA considers power sources early in its mission review process. Multiple depleted in the 2020s, according to NASA and DOE officials and factors could affect NASA’s demand for RPS and Pu-238. For example, high documentation. With NASA funding, costs associated with RPS and missions can affect the demand for RPS DOE initiated the Pu-238 Supply because, according to officials, NASA’s budget can only support one RPS Project in 2011, with a goal of mission about every 4 years. -
Cosmos: a Spacetime Odyssey (2014) Episode Scripts Based On
Cosmos: A SpaceTime Odyssey (2014) Episode Scripts Based on Cosmos: A Personal Voyage by Carl Sagan, Ann Druyan & Steven Soter Directed by Brannon Braga, Bill Pope & Ann Druyan Presented by Neil deGrasse Tyson Composer(s) Alan Silvestri Country of origin United States Original language(s) English No. of episodes 13 (List of episodes) 1 - Standing Up in the Milky Way 2 - Some of the Things That Molecules Do 3 - When Knowledge Conquered Fear 4 - A Sky Full of Ghosts 5 - Hiding In The Light 6 - Deeper, Deeper, Deeper Still 7 - The Clean Room 8 - Sisters of the Sun 9 - The Lost Worlds of Planet Earth 10 - The Electric Boy 11 - The Immortals 12 - The World Set Free 13 - Unafraid Of The Dark 1 - Standing Up in the Milky Way The cosmos is all there is, or ever was, or ever will be. Come with me. A generation ago, the astronomer Carl Sagan stood here and launched hundreds of millions of us on a great adventure: the exploration of the universe revealed by science. It's time to get going again. We're about to begin a journey that will take us from the infinitesimal to the infinite, from the dawn of time to the distant future. We'll explore galaxies and suns and worlds, surf the gravity waves of space-time, encounter beings that live in fire and ice, explore the planets of stars that never die, discover atoms as massive as suns and universes smaller than atoms. Cosmos is also a story about us. It's the saga of how wandering bands of hunters and gatherers found their way to the stars, one adventure with many heroes. -
1 the New Horizons Spacecraft Glen H. Fountain, David Y
The New Horizons Spacecraft Glen H. Fountain, David Y. Kusnierkiewicz, Christopher B. Hersman, Timothy S. Herder, Thomas B Coughlin, William T. Gibson, Deborah A. Clancy, Christopher C. DeBoy, T. Adrian Hill, James D. Kinnison, Douglas S. Mehoke, Geffrey K. Ottman, Gabe D. Rogers, S. Alan Stern, James M. Stratton, Steven R. Vernon, Stephen P. Williams Abstract The New Horizons spacecraft was launched on January 19, 2006. The spacecraft was designed to provide a platform for the seven instruments designated by the science team to collect and return data from Pluto in 2015 that would meet the requirements established by the National Aeronautics and Space Administration (NASA) Announcement of Opportunity AO-OSS-01. The design drew on heritage from previous missions developed at The Johns Hopkins University Applied Physics Laboratory (APL) and other NASA missions such as Ulysses. The trajectory design imposed constraints on mass and structural strength to meet the high launch acceleration consistent with meeting the AO requirement of returning data prior to the year 2020. The spacecraft subsystems were designed to meet tight resource allocations (mass and power) yet provide the necessary control and data handling finesse to support data collection and return when the one way light time during the Pluto fly-by is 4.5 hours. Missions to the outer regions of the solar system (where the solar irradiance is 1/1000 of the level near the Earth) require a Radioisotope Thermoelectric Generator (RTG) to supply electrical power. One RTG was available for use by New Horizons. To accommodate this constraint, the spacecraft electronics were designed to operate on less than 200 W. -
The Radioscience Experiment on New Horizons
The Radioscience Experiment on New Horizons Ivan R. Linscott(1), Michael K. Bird(2), David P. Hinson(1)(3), Martin Pätzold(2), and G. Lenard Tyler(1) (1)Stanford University,350 Serra Mall, Stanford, CA, 94305, [email protected] [email protected], [email protected], [email protected], [email protected] (2)Rhenish Institute for Environmental Research University of Cologne, Germany, (3)SETI Institute Abstract REX is the Radioscience Experiment in the payload on the New Horizons spacecraft en-route to its encounter with Pluto in July of 2015. REX will obtain the temperature and pressure profiles of Pluto's tenuous atmosphere while measuring radiometric temperature, gravitational moments and ionosphere structure. Additional targets of opportunity, at lower priority, include the search for an atmosphere at Charon, improved gravity precision and a bistatic surface scattering on Pluto. For all but radiometry, these measurements take advantage of a high-power, X- band uplink transmitted from the earth, received on the spacecraft with the aid of a high-gain antenna, a low-noise X-band receiver and an ultrastable oscillator (USO), as a frequency reference. This combination enables REX to sense Pluto's atmosphere with precision of ~ 0.1 Pa (1 μbar), and ~ 3 K, and a neutral number density of 4x1019/m3. Critical measurement techniques will be validated using results from the May 20, 2011, Lunar Occultation of New Horizons spacecraft. 1. Introduction New Horizons is a NSAS mission to Pluto launched on January 2006, with a nine year cruise and intended encounter with Pluto on July 14, 2015. -
NASA Finds Neptune Moons Locked in 'Dance of Avoidance' 15 November 2019, by Gretchen Mccartney
NASA finds Neptune moons locked in 'dance of avoidance' 15 November 2019, by Gretchen McCartney Although the dance may appear odd, it keeps the orbits stable, researchers said. "We refer to this repeating pattern as a resonance," said Marina Brozovi?, an expert in solar system dynamics at NASA's Jet Propulsion Laboratory in Pasadena, California, and the lead author of the new paper, which was published Nov. 13 in Icarus. "There are many different types of 'dances' that planets, moons and asteroids can follow, but this one has never been seen before." Far from the pull of the Sun, the giant planets of the Neptune Moon Dance: This animation illustrates how the outer solar system are the dominant sources of odd orbits of Neptune's inner moons Naiad and gravity, and collectively, they boast dozens upon Thalassa enable them to avoid each other as they race dozens of moons. Some of those moons formed around the planet. Credit: NASA alongside their planets and never went anywhere; others were captured later, then locked into orbits dictated by their planets. Some orbit in the opposite direction their planets rotate; others swap orbits Even by the wild standards of the outer solar with each other as if to avoid collision. system, the strange orbits that carry Neptune's two innermost moons are unprecedented, according to Neptune has 14 confirmed moons. Neso, the newly published research. farthest-flung of them, orbits in a wildly elliptical loop that carries it nearly 46 million miles (74 million Orbital dynamics experts are calling it a "dance of kilometers) away from the planet and takes 27 avoidance" performed by the tiny moons Naiad years to complete. -
David J. Mccomas - Refereed Publications
David J. McComas - Refereed Publications 1982 1. McComas, D.J. and S.J. Bame, Radially Uniform Electron Source, Rev. of Sci. Instrum., 53, 1490-1491, 1982. 2. Feldman, W.C., S.J. Bame, S.P. Gary, J.T. Gosling, D.J. McComas, M.F. Thomsen, G. Paschmann, N. Sckopke, M.M. Hoppe, C.T. Russell, Electron Heating Within the Earth's Bowshock, Phys. Rev. Lett., 49, 199-201,1982. 1983 3. Feldman, W.C., R.C. Anderson, S.J. Bame, S.P. Gary, J.T. Gosling, D.J. McComas, M.F. Thomsen, G. Paschmann, M.M. Hoppe, Electron Velocity Distributions Near the Earth's Bowshock, J. Geophys. Res., 88, 96-110, 1983. 4. Bame, S.J., R.C. Anderson, J.R. Asbridge, D.N. Baker, W.C. Feldman, J.T. Gosling, E.W. Hones, Jr., D.J. McComas, R.D. Zwickl, Plasma Regimes in the Deep Geomagnetic Tail: ISEE-3, Geophys. Res. Lett., 10, 912-915, 1983. 1984 5. McComas, D.J. and S.J. Bame, Channel Multiplier Compatible MaterialsLifetime Tests, Rev. Sci. Inst., 55, 463-467, 1984. 6. Hones, Jr., E.W., D.N. Baker, S.J. Bame, W.C. Feldman, J.T. Gosling, D.J. McComas, R.D. Zwickl, J.A. Slavin., E.J. Smith, B.T. Tsurutani, Structure of the Magnetotail at 220RE its Response to Geomagnetic Activity, Geophys. Res. Lett., 11, 5-7, 1984. 7. Baker, D.N., S.J. Bame, W.C. Feldman, J.T. Gosling, P.R. Higbie, E.W. Hones, Jr., D.J. McComas, R.D. Zwickl, Correlated Dynamical Changes in the Near Earth and Distant Magnetotail Regions: ISEE-3, J. -
New Horizons 2 Alan Stern (Swri), Rick Binzel (MIT), Hal Levison
New Horizons 2 Alan Stern (SwRI), Rick Binzel (MIT), Hal Levison (SwRI), Rosaly Lopes (JPL), Bob Millis (Lowell Observatory), and Jeff Moore (NASA Ames) New Horizons is the inaugural mission in NASA’s New Frontiers program—a series of mid-sized planetary exploration projects. This mission was competitively selected in 2001 after a peer review competition between industry-university teams. The mission is on track toward a planned launch in January 2006—just over 6 months hence. The primary objective of New Horizons (NH) is to make the first reconnaissance of the solar system’s farthest planet, Pluto, its comparably sized satellite Charon. If an extended mission is approved, New Horizons may be able to also flyby a Kuiper Belt Object (KBOs) farther from the Sun. The exploration of the Kuiper Belt and Pluto-Charon was ranked as the highest new start priority for planetary exploration by the National Research Council’s recently completed (2002) Decadal Survey for Planetary Science. In accomplishing its goals, the mission is expected to reveal fundamental new insights into the nature of the outer solar system, the formation history of the planets, the workings of binary worlds, and the ancient repository of water and organic building blocks called the Kuiper Belt. Beyond its scientific ambitions, New Horizons is also breaking ground in lowering the cost of exploration of the outer solar system—for it is being built and launched for what are literally dimes on the dollar compared to deep outer solar system missions like Voyager, Galileo, and Cassini. The New Horizons spacecraft carries a suite of seven advanced, miniaturized instruments to obtain detailed imagery, mapping spectroscopy, thermal mapping, gravitational data, and in situ plasma composition, density, and energy sampling of the exotic, icy Pluto- Charon binary and a modest-sized (~50 km diameter) KBO.