Information to Users
Total Page:16
File Type:pdf, Size:1020Kb
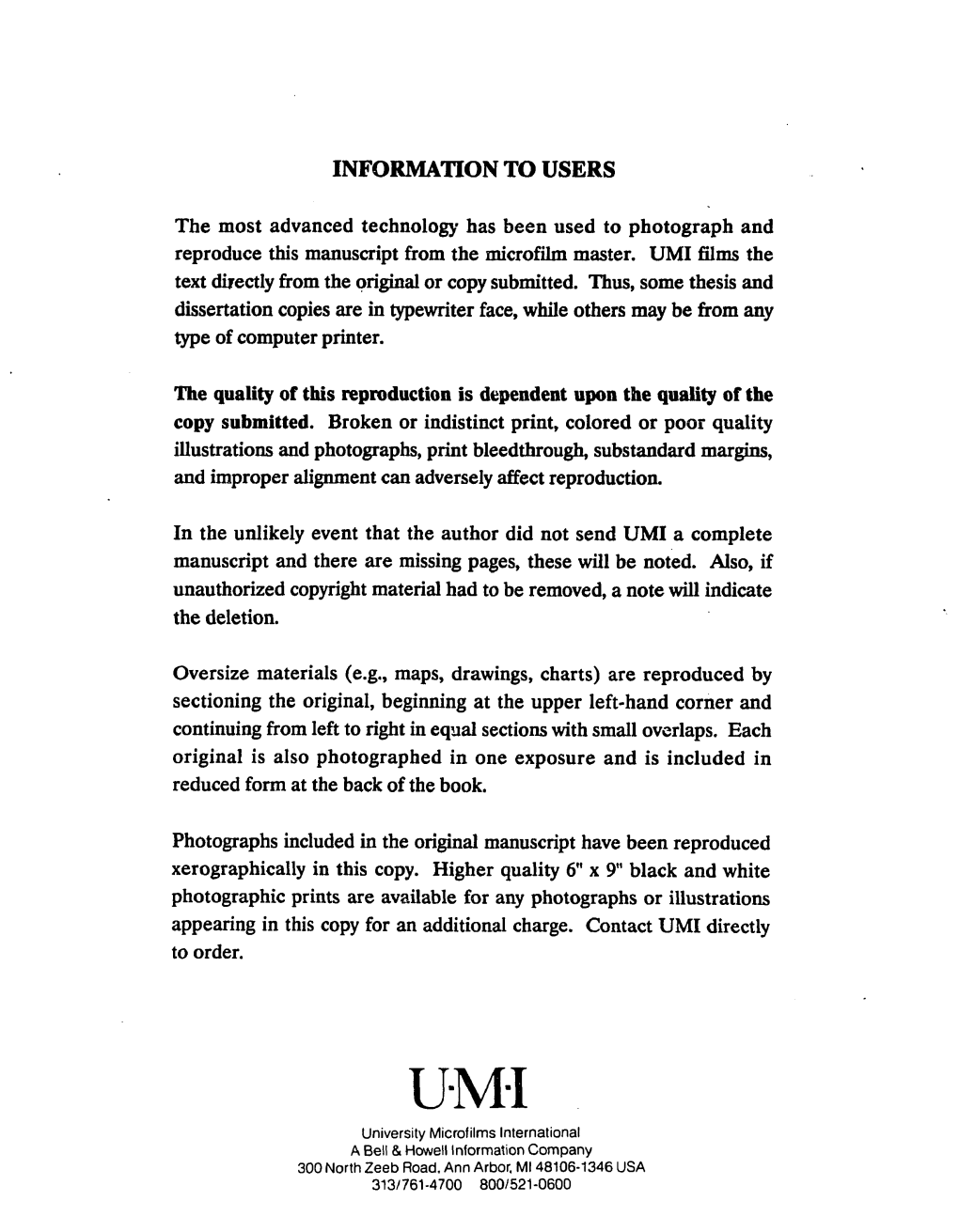
Load more
Recommended publications
-
To Identify Irregularities in O-Cdiagrams with "Changes Of
VOL. 23, 1937 ASTRONOMY: STERNE AND CAMPBELL 115 CHANGES OF PERIOD IN VARIABLE STARS OF LONG PERIOD By T. E. STERNE AND L. CAMPBELL THE HARVARD COLLEGE OBSERVATORY Communicated December 23, 1936 One of us has investigated elsewhere' the mathematical theory of the accuracy of periods determined from dates of maximum, or minimum, or mth magnitude increasing or diminishing, of a variable star. The existence of cumulative errors2 in the periods of long-period variables was shown to provide a simple explanation for the major part of their observed irregu- larities of period, as revealed for instance by O-C diagrams,3 and plots of cycles against epoch number. The existence of cumulative errors was shown to be in itself sufficient to cause an erratic behavior in O-C diagrams, so that such diagrams did not by themselves furnish safe evidence for changes of true, or mean, period. Further, it was shown that a value of the mean period, obtained from observations extending over any finite interval of time, would be liable to errors of sampling, so that the mere existence of a scatter among' values of the mean period obtained from differing intervals of time was not in itself evidence for a changing true or mean period. Before the appearance of the previously mentioned investigations, it had been the common practice among workers in the field of variable stars to identify irregularities in O-C diagrams with "changes of period" in the stars. Thus, of the 566 variables with known long periods listed4 in the Katalog der Elemente des Lichtwechsels fur 1687 verdnderliche Sterne, sine terms were assigned to 85. -
The Winds of Cataclysmic Variables
View metadata, citation and similar papers at core.ac.uk brought to you by CORE provided by CERN Document Server 1997, in Cosmic Winds and the Heliosphere, ed. J. R. Jokipii, C. P. Sonett, & M. S. Giampapa (Tucson: Univ. of Arizona Press) THE WINDS OF CATACLYSMIC VARIABLES CHRISTOPHER W. MAUCHE Lawrence Livermore National Laboratory and JOHN C. RAYMOND Harvard-Smithsonian Center for Astrophysics We present an observational and theoretical review of the winds of cata- clysmic variables (CVs). Specifically, we consider the related problems of the geometry, ionization state, and mass-loss rate of the winds of CVs. We present evidence of wind variability and discuss the results of studies of eclipsing CVs. Finally, we consider the properties of accretion disk wind and magnetic wind models. Some of these models predict substantial angular momentum loss, which could affect both disk structure and binary evolution. I. Introduction Cataclysmic variables (CVs) are a large, diverse class of short- period semi-detached mass-exchanging binaries composed of a white dwarf, a late-type (G, K, or M) main-sequence secondary, and, typi- cally, an accretion disk. The various CV subtypes include novae, which are powered by the thermonuclear burning of material on the surface of the white dwarf, and dwarf novae and nova-like variables, which are powered by the accretion of material transferred from the secondary to the white dwarf. For recent reviews of CVs, refer to Cropper (1990), Livio (1994), and C´ordova (1995). Among the nova-like variables, there exists a class in which the magnetic field of the white dwarf is strong enough to influence the flow of material accreted by the white dwarf. -
Meteor.Mcse.Hu
MCSE 2016/3 meteor.mcse.hu A Mars négy arca SZJA 1%! Az MCSE adószáma: 19009162-2-43 A Tharsis-régió három pajzsvulkánja és az Olympus Mons a Mars Express 2014. június 29-én készült felvételén (ESA / DLR / FU Berlin / Justin Cowart). TARTALOM Áttörés a fizikában......................... 3 GW150914: elõször hallottuk az Univerzum zenéjét....................... 4 A csillagászat ............................ 8 meteorA Magyar Csillagászati Egyesület lapja Journal of the Hungarian Astronomical Association Csillagászati hírek ........................ 10 H–1300 Budapest, Pf. 148., Hungary 1037 Budapest, Laborc u. 2/C. A távcsövek világa TELEFON/FAX: (1) 240-7708, +36-70-548-9124 Egy „klasszikus” naptávcsõ születése ........ 18 E-MAIL: [email protected], Honlap: meteor.mcse.hu HU ISSN 0133-249X Szabadszemes jelenségek Kiadó: Magyar Csillagászati Egyesület Gyöngyházfényû felhõk – történelmi észlelés! .. 22 FÔSZERKESZTÔ: Mizser Attila A hónap asztrofotója: hajnali együttállás ....... 27 SZERKESZTÔBIZOTTSÁG: Dr. Fûrész Gábor, Dr. Kiss László, Dr. Kereszturi Ákos, Dr. Kolláth Zoltán, Bolygók Mizser Attila, Dr. Sánta Gábor, Sárneczky Krisztián, Mars-oppozíció 2014 .................... 28 Dr. Szabados László és Dr. Szalai Tamás SZÍNES ELÕKÉSZÍTÉS: KÁRMÁN STÚDIÓ Nap FELELÔS KIADÓ: AZ MCSE ELNÖKE Téli változékony Napok .................38 A Meteor elôfizetési díja 2016-ra: (nem tagok számára) 7200 Ft Hold Egy szám ára: 600 Ft Januári Hold .........................42 Az egyesületi tagság formái (2016) • rendes tagsági díj (jogi személyek számára is) Meteorok -
The Identification of Hydrogen-Deficient Cataclysmic
The Identification of Hydrogen-Deficient Cataclysmic Variable Donor Stars1;2 Thomas E. Harrison Department of Astronomy, New Mexico State University, Box 30001, MSC 4500, Las Cruces, NM 88003-8001 Received ; accepted arXiv:1806.04612v1 [astro-ph.SR] 12 Jun 2018 { 2 { ABSTRACT We have used ATLAS12 to generate hydrogen-deficient stellar atmospheres to allow us to construct synthetic spectra to explore the possibility that the donor stars in some cataclysmic variables (CVs) are hydrogen deficient. We find that four systems, AE Aqr, DX And, EY Cyg, and QZ Ser have significant hydrogen deficits. We confirm that carbon and magnesium deficits, and sodium enhancements, are common among CV donor stars. The three Z Cam systems we observed are found to have solar metallicities and no abundance anomalies. Two of these objects, Z Cam and AH Her, have M-type donor stars; much cooler than expected given their long orbital periods. By using the combination of equivalent width measurements and light curve modeling, we have developed the ability to account for contamination of the donor star spectra by other luminosity sources in the binary. This enables more realistic assessments of secondary star metallicities. We find that the use of equivalent width measurements should allow for robust metallicities and abundance anomalies to be determined for CVs with M-type donor stars. Key words: stars | infrared: stars | stars: novae, cataclysmic variables | stars: abundances | stars: individual (DX And, RX And, AE Aqr, Z Cam, SY Cnc, EM Cyg, EY Cyg, SS Cyg, V508 Dra, AH Her, RU Peg, GK Per, QZ Ser) 1Partially based on observations obtained with the Apache Point Observatory 3.5-meter telescope, which is owned and operated by the Astrophysical Research Consortium. -
Dwarf Nova-Type Cataclysmic Variable Stars Are Significant Radio Emitters
MNRAS 000,1{13 (2016) Preprint 14 November 2018 Compiled using MNRAS LATEX style file v3.0 Dwarf nova-type cataclysmic variable stars are significant radio emitters Deanne L. Coppejans,1? Elmar. G. K¨ording,1 James C.A. Miller-Jones,2 Michael P. Rupen,3 Gregory R. Sivakoff,4 Christian Knigge,5 Paul J. Groot,1 Patrick A. Woudt,6 Elizabeth O. Waagen7 and Matthew Templeton 1Department of Astrophysics/IMAPP, Radboud University, P.O. Box 9010, 6500 GL Nijmegen, The Netherlands 2International Centre for Radio Astronomy Research, Curtin University, GPO Box U1987, Perth, WA 6845, Australia 3National Research Council of Canada, Herzberg Astronomy and Astrophysics, Dominion Radio Astrophysical Observatory, P.O. Box 248, Penticton, BC V2A 6J9, Canada 4Department of Physics, University of Alberta, CCIS 4-183, Edmonton, Alberta T6G 2E1, Canada 5School of Physics and Astronomy, Southampton University, Highfield, Southampton SO17 1BJ, UK 6Department of Astronomy, University of Cape Town, Private Bag X3, 7701 Rondebosch, South Africa 7American Association of Variable Star Observers, 49 Bay State Road, Cambridge, MA 02138, USA Accepted XXX. Received YYY; in original form ZZZ ABSTRACT We present 8{12 GHz radio light curves of five dwarf nova (DN) type Cataclysmic Variable stars (CVs) in outburst (RX And, U Gem and Z Cam), or superoutburst (SU UMa and YZ Cnc), increasing the number of radio-detected DN by a factor of two. The observed radio emission was variable on time-scales of minutes to days, and we argue that it is likely to be synchrotron emission. This sample shows no correlation between the radio luminosity and optical luminosity, orbital period, CV class, or outburst type; however higher-cadence observations are necessary to test this, as the measured luminosity is dependent on the timing of the observations in these variable objects. -
Index to Volume 44
202 Index, JAAVSO Volume 44, 2016 Index to Volume 44 Crast, Jack, and George Silvis Eggen Card Project: Progress and Plans (Abstract) 200 Author de Ponthière, Pierre, and Franz-Josef (Josch) Hambsch, Kenneth Menzies, Richard Sabo Allen, Chris, in Thomas Karlsson et al. TU Comae Berenices: Blazhko RR Lyrae Star in a Potential 50 Forgotten Miras 156 Binary System 18 Alton, Kevin B. Deibert, Emily, and John R. Percy CCD Photometry and Roche Modeling of the Eclipsing Overcontact Studies of the Long Secondary Periods in Pulsating Red Giants 94 Binary Star System TYC 01963-0488-1 87 Dempsey, Frank Amaral, Ariel, and John R. Percy Why are the Daily Sunspot Observations Interesting? One Observer’s An Undergraduate Research Experience on Studying Variable Stars 72 Perspective (Abstract) 83 Anon. Denig, William F. Index to Volume 44 202 Impacts of Extended Periods of Low Solar Activity on Artusi, Elisabetta, and Giancarlo Conselvan, Antonio Tegon, Climate (Abstract) 83 Danilo Zardin Dose, Eric Crowded Fields Photometry with DAOPHOT 149 First Look at Photometric Reduction via Mixed-Model Regression Axelsen, Roy Andrew (Poster abstract) 198 Erratum. Current Light Elements of the δ Scuti Star Evich, Alexander, and Howard D. Mooers, William S. Wiethoff V393 Carinae 201 Monitoring the Continuing Spectral Evolution of Nova Delphini 2013 Axelsen, Roy Andrew, and Tim Napier-Munn (V339 Del) with Low Resolution Spectroscopy 60 The High Amplitude δ Scuti Star AD Canis Minoris 119 Faulkner, Danny R., in Ronald G. Samec et al. Bell, Ellyn, in Barton J. Pritzl et al. First Photometric Analysis of the Solar-Type Binary, V428 RR Lyrae in Sagittarius Dwarf Globular Clusters (Poster abstract) 198 (NSV 395), in the field of NGC 188 101 Bengtsson, Hans, in Thomas Karlsson et al. -
General Bibliography
General Bibliography These are selected, recently published books on the history of astronomy (excluding textbooks). Consult them in addition to article, Selected References. Preference is given to those books written in–or translated into–English, the language of this encyclopedia. References to biographies, institutional histories, conference procee- dings, and journal/compendium articles appear in individual entry bibliographies. Ince, Martin. Dictionary of Astronomy. Fitzroy Dearborn, 1997. RENAISSANCE ASTRONOMY GENERAL Crowe, Michael J. Theories of the World from Antiquity to the Copernican Revolu- Abetti, Giorgio. The History of Astronomy. H. Schuman, 1952. tion. Dover Publications, 1990. Berry, Arthur. A Short History of Astronomy from Earliest Times Through the Eleanor-Roos, Anna Marie. Luminaries in the Natural World: The Sun and Moon Nineteenth Century. Dover Publications, 1961. in England, 1400–1720. Peter Lang Publishing, 2001. Clerke, Agnes. A Popular History of Astronomy During the Nineteenth Century. Jervis, Jane. Cometary Theory in Fifteenth-Century Europe. D. Reidel Publishing A. and C. Black, 1902. Company, 1985. DeVorkin, David (editor). Beyond Earth: Mapping the Universe. National Geo- Koyré, Alexander. The Astronomical Revolution: Copernicus – Kepler – Borrelli. graphic Society, 2002. Dover Publications, 1992. Ferris, Timothy. Coming of Age in the Milky Way. William Morrow & Company, Kuhn, Thomas S. The Copernican Revolution: Planetary Astronomy in the 1988. Development of Western Thought. Harvard University Press, 1957. Grant, Robert. History of Physical Astronomy From the Earliest Ages to the Middle Randles, W. G. L. The Unmaking of the Medieval Christian Cosmos, 1500–1760: of the Nineteenth Century. Johnson Reprint Corporation, 1966. From Solid Heavens to Boundless Æther. Ashgate Publishing Company, Hoskin, Michael. -
Multi-Wavelength Accretion Studies of Cataclysmic Variable Stars
Multi-wavelength Accretion Studies of Cataclysmic Variable Stars Proefschrift ter verkrijging van de graad van doctor aan de Radboud Universiteit Nijmegen op gezag van de rector magnificus prof. dr. J.H.J.M. van Krieken, volgens besluit van het college van decanen in het openbaar te verdedigen op Maandag 3 Oktober 2016 om 10:30 uur precies door Deanne Larissa Coppejans geboren op 7 Februari 1988 te Zuid-Afrika Promotoren: Prof. dr. P.J. Groot Prof. dr. P.A. Woudt University of Cape Town, SA Copromotor: Dr. E.G. Körding Manuscriptcommissie: Prof. dr. R. Kleiss Prof. dr. F.W.M. Verbunt Prof. dr. R.P. Fender University of Oxford, UK Prof. dr. A. Achterberg Dr. S. Scaringi MPE, Duitsland c 2016, Deanne Larissa Coppejans Multi-wavelength Accretion Studies of Cataclysmic Variable Stars Thesis, Radboud University Nijmegen Printed by Ipskamp Printing Illustrated; with bibliographic information and Dutch summary ISBN: 978-94-028-0320-4 Contents 1 Introduction 1 1.1 Cataclysmic Variables..................................1 1.1.1 Structure.....................................1 1.1.2 Mass Transfer...................................3 1.1.3 Formation and Evolution............................4 1.2 Dwarf Novae and Novalikes...............................6 1.2.1 Dwarf Nova Outburst Mechanism........................7 1.2.2 Superoutburst Mechanism............................9 1.3 Radio Emission from Cataclysmic Variables...................... 10 2 High-speed photometry of faint Cataclysmic Variables 13 2.1 Introduction........................................ 14 2.2 Observations....................................... 15 2.3 Discussion and Conclusions............................... 40 3 Statistical properties of dwarf novae-type Cataclysmic Variables 43 3.1 Introduction........................................ 44 3.2 CRTS........................................... 45 3.3 Classification Script................................... 47 3.4 The Outburst Catalogue................................ -
ABUNDANCE DERIVATIONS for the SECONDARY STARS in CATACLYSMIC VARIABLES from NEAR-INFRARED SPECTROSCOPY Thomas E
The Astrophysical Journal, 833:14 (30pp), 2016 December 10 doi:10.3847/0004-637X/833/1/14 © 2016. The American Astronomical Society. All rights reserved. ABUNDANCE DERIVATIONS FOR THE SECONDARY STARS IN CATACLYSMIC VARIABLES FROM NEAR-INFRARED SPECTROSCOPY Thomas E. Harrison1,2 Department of Astronomy, New Mexico State University, Box 30001, MSC 4500, Las Cruces, NM 88003-8001, USA; [email protected] Received 2016 August 3; revised 2016 September 2; accepted 2016 September 29; published 2016 December 1 ABSTRACT We derive metallicities for 41 cataclysmic variables (CVs) from near-infrared spectroscopy. We use synthetic spectra that cover the 0.8 μmλ2.5μm bandpass to ascertain the value of [Fe/H] for CVs with K-type donors, while also deriving abundances for other elements. Using calibrations for determining [Fe/H] from the K- band spectra of M-dwarfs, we derive more precise values for Teff for the secondaries in the shortest period CVs, and examine whether they have carbon deficits. In general, the donor stars in CVs have subsolar metallicities. We confirm carbon deficits for a large number of systems. CVs with orbital periods >5 hr are most likely to have unusual abundances. We identify four CVs with CO emission. We use phase-resolved spectra to ascertain the mass and radius of the donor in U Gem. The secondary star in U Gem appears to have a lower apparent gravity than a main sequence star of its spectral type. Applying this result to other CVs, we find that the later-than-expected spectral types observed for many CV donors are mostly an effect of inclination. -
The COLOUR of CREATION Observing and Astrophotography Targets “At a Glance” Guide
The COLOUR of CREATION observing and astrophotography targets “at a glance” guide. (Naked eye, binoculars, small and “monster” scopes) Dear fellow amateur astronomer. Please note - this is a work in progress – compiled from several sources - and undoubtedly WILL contain inaccuracies. It would therefor be HIGHLY appreciated if readers would be so kind as to forward ANY corrections and/ or additions (as the document is still obviously incomplete) to: [email protected]. The document will be updated/ revised/ expanded* on a regular basis, replacing the existing document on the ASSA Pretoria website, as well as on the website: coloursofcreation.co.za . This is by no means intended to be a complete nor an exhaustive listing, but rather an “at a glance guide” (2nd column), that will hopefully assist in choosing or eliminating certain objects in a specific constellation for further research, to determine suitability for observation or astrophotography. There is NO copy right - download at will. Warm regards. JohanM. *Edition 1: June 2016 (“Pre-Karoo Star Party version”). “To me, one of the wonders and lures of astronomy is observing a galaxy… realizing you are detecting ancient photons, emitted by billions of stars, reduced to a magnitude below naked eye detection…lying at a distance beyond comprehension...” ASSA 100. (Auke Slotegraaf). Messier objects. Apparent size: degrees, arc minutes, arc seconds. Interesting info. AKA’s. Emphasis, correction. Coordinates, location. Stars, star groups, etc. Variable stars. Double stars. (Only a small number included. “Colourful Ds. descriptions” taken from the book by Sissy Haas). Carbon star. C Asterisma. (Including many “Streicher” objects, taken from Asterism. -
Download Full-Text
International Journal of Astronomy 2020, 9(1): 3-11 DOI: 10.5923/j.astronomy.20200901.02 The Processes that Determine the Formation and Chemical Composition of the Atmosphere of the Body in Orbit Weitter Duckss Independent Researcher, Zadar, Croatia Abstract The goal of this article is to analyze the formation of an atmosphere on the orbiting planets and to determine the processes that participate in the formation of an atmospheric chemical composition, as well as in determining it. The research primarily analyzes the formation of atmospheres on the objects of different sizes (masses) and at the same or different orbital distances. This paper analyzes the influence of a star's temperature, the space and the orbit's distance to an object's temperature level, as well as the influence of the operating temperature of atoms and chemical compounds to chemical composition and the representation of elements and compounds in an atmosphere. The objects, which possess different masses and temperatures, are able to create and do create different compositions and sizes of atmospheres in the same or different distances from their main objects (Saturn/Titan or Pluto). The processes that are included in the formation of an atmosphere are the following: operating temperatures of compounds and atoms, migrations of hydrogen, helium and the other elements and compounds towards a superior mass. The lack of oxygen and hydrogen is additionally related to the level of temperature of space, which can be classified into internal (characterized by the lack of hydrogen) and the others (characterized by the lack of oxygen). Keywords Atmosphere, Chemical composition of the atmosphere, Migration of the atmosphere the atmosphere even though they are not in a gaseous state at 1. -
Mentor Astro Test 2009
1 Team Name_________________________________________ Team Number_______________ Possibly Useful Information RR Lyrae stars have Mv=0.6 The period‐luminosity relationship for Classical Cepheid is given by Where P is given in days Data for the Sun Mass 2 x 1030 kg Radius 7 x 105 km Luminosity 3.8 x 1026 W Temperature = 5778 K Spectral Type G2V Absolute visual magnitude MV = 4.83 1 Astronomical Unit 1.5 x 108 km DSO’s for this year Circinus X‐1 RU Virginis Epsilon Aurigae RX Andromedae Z Andromedae SN 1006 RX J0822‐4300 G292.0+1.8 NGC 2440 Betelgeuse RS Ophiuchi Mira T Tauri RS Puppis Hinds Variable Nebula 2 Team Name_________________________________________ Team Number_______________ Point values for the problems 1a 6 3b 2 1b 4 3c 4 1c 6 3d 10 1d 6 4a 2 1e 6 4b 2 1f 6 4c 6 1g 2 5a 2 1h 2 5b 2 1i 15 5c 4 2a 2 5d 5 2b 4 5e 2 2c 2 6a 2 2d 2 6b 2 2e 4 6c 4 2f 8 6d 4 3a 2 Total Points 130 3 Team Name_________________________________________ Team Number_______________ 1 This is a picture of one of this year's DSOs, α Orionis or Betelgeuse. For the purpose of this exercise we will assume: This picture is taken in the V band, in spite of the color. The scale bar gives the measured diameter of the object as 60 milli arc seconds (mas). The apparent V magnitude of the object was measured on the day the picture was obtained mV=0.58.