Benchmark Thermochemistry of the C Nh {2N+ 2} Alkane Isomers (N
Total Page:16
File Type:pdf, Size:1020Kb
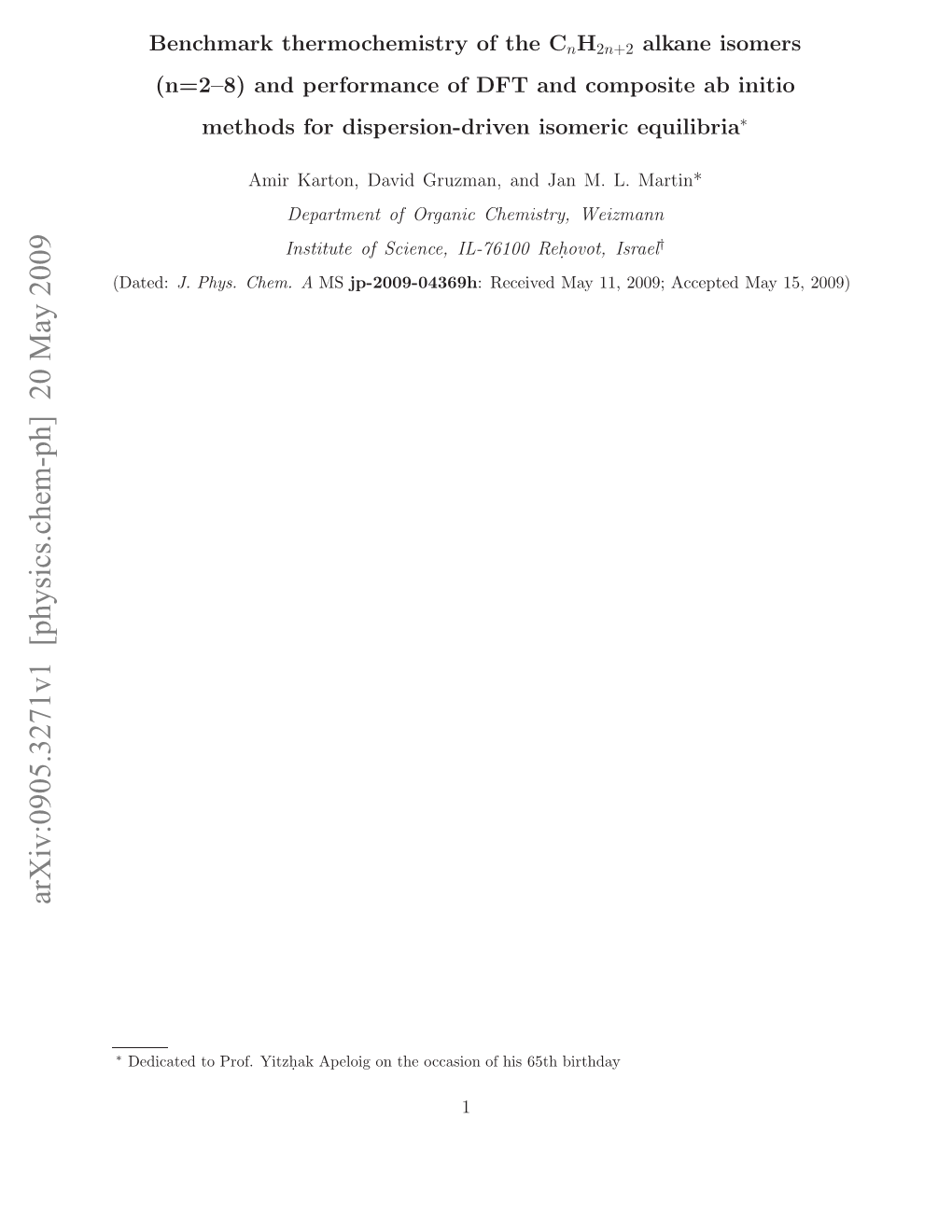
Load more
Recommended publications
-
Review of Market for Octane Enhancers
May 2000 • NREL/SR-580-28193 Review of Market for Octane Enhancers Final Report J.E. Sinor Consultants, Inc. Niwot, Colorado National Renewable Energy Laboratory 1617 Cole Boulevard Golden, Colorado 80401-3393 NREL is a U.S. Department of Energy Laboratory Operated by Midwest Research Institute • Battelle • Bechtel Contract No. DE-AC36-99-GO10337 May 2000 • NREL/SR-580-28193 Review of Market for Octane Enhancers Final Report J.E. Sinor Consultants, Inc. Niwot, Colorado NREL Technical Monitor: K. Ibsen Prepared under Subcontract No. TXE-0-29113-01 National Renewable Energy Laboratory 1617 Cole Boulevard Golden, Colorado 80401-3393 NREL is a U.S. Department of Energy Laboratory Operated by Midwest Research Institute • Battelle • Bechtel Contract No. DE-AC36-99-GO10337 NOTICE This report was prepared as an account of work sponsored by an agency of the United States government. Neither the United States government nor any agency thereof, nor any of their employees, makes any warranty, express or implied, or assumes any legal liability or responsibility for the accuracy, completeness, or usefulness of any information, apparatus, product, or process disclosed, or represents that its use would not infringe privately owned rights. Reference herein to any specific commercial product, process, or service by trade name, trademark, manufacturer, or otherwise does not necessarily constitute or imply its endorsement, recommendation, or favoring by the United States government or any agency thereof. The views and opinions of authors expressed herein do not necessarily state or reflect those of the United States government or any agency thereof. Available electronically at http://www.doe.gov/bridge Available for a processing fee to U.S. -
APPENDIX M SUMMARY of MAJOR TYPES of Carfg2 REFINERY
APPENDIX M SUMMARY OF MAJOR TYPES OF CaRFG2 REFINERY MODIFICATIONS Appendix M: Summan/ of Major Types of CaRFG2 Refinery Modifications: Alkylation Units A process unit that combines small-molecule hydrocarbon gases produced in the FCCU with a branched chain hydrocarbon called isobutane, producing a material called alkylate, which is blended into gasoline to raise the octane rating. Alkylate is a high octane, low vapor pressure gasoline blending component that essentially contains no olefins, aromatics, or sulfur. This plant improves the ultimate gasoline-making ability of the FCC plant. Therefore, many California refineries built new or modified existing units to increase alkylate production to blend and to produce greater amounts of CaRFG2. Alkylate is produced by combining C3, C4, and C5 components with isobutane (nC4). The process of alkylation is the reverse of cracking. Olefins (such as butenes and propenes) and isobutane are used as feedstocks and combined to produce alkylate. This process enables refiners to utilize lighter components that otherwise could not be blended into gasoline due to their high vapor pressures. Feed to alkylation unit can include pentanes from light cracked gasoline treaters, isobutanes from butane isomerization unit, and C3/C4 streams from delayed coking units. Isomerization Units - C4/C5/C6 A refinery that has an alkylation plant is not likely to have exactly enough is-butane to match the proplylene and butylene (olefin) feeds. The refiner usually has two choices - buy iso-butane or make it in a butane isomerization (Bl) plant. Isomerization is the rearrangement of straight chain hydrocarbon molecules to form branched chain products or to convert normal paraffins to their isomer. -
Chemical Kinetic Research on HCCI & Diesel Fuels
Lawrence Livermore National Laboratory Chemical Kinetic Research on HCCI & Diesel Fuels William J. Pitz (PI), Charles K. Westbrook, Marco Mehl, M. Lee Davisson Lawrence Livermore National Laboratory May 12, 2009 Project ID # ace_13_pitz DOE National Laboratory Advanced Combustion Engine R&D Merit Review and Peer Evaluation Washington, DC This presentation does not contain any proprietary or confidential information This work performed under the auspices of the U.S. Department of Energy by LLNL-PRES-411416 Lawrence Livermore National Laboratory under Contract DE-AC52-07NA27344 Overview Timeline Barriers • Ongoing project with yearly • Increases in engine efficiency and direction from DOE decreases in engine emissions are being inhibited by an inadequate ability to simulate in-cylinder combustion and emission formation processes • Chemical kinetic models are critical for Budget improved engine modeling and ultimately for engine design • Funding received in FY08 and expected in FY09: Partners • 800K (DOE) • Interactions/ collaborations: DOE Working Group, National Labs, many universities, FACE Working group. Lawrence Livermore National Laboratory 2 LLNL-PRES-411416LLNL-PRES- 411416 2009 DOE Merit Review Relevance to DOE objectives Support DOE objectives of petroleum-fuel displacement by • Improving engine models so that further improvements in engine efficiency can be made • Developing models for alternative fuels (biodiesel, ethanol, other biofuels, oil-sand derived fuels, Fischer-Tropsch derived fuels) so that they can be better used -
1 Refinery and Petrochemical Processes
3 1 Refinery and Petrochemical Processes 1.1 Introduction The combination of high demand for electric cars and higher automobile engine effi- ciency in the future will mean less conversion of petroleum into fuels. However, the demand for petrochemicals is forecast to rise due to the increase in world popula- tion. With this, it is expected that modern and more innovative technologies will be developed to serve the growth of the petrochemical market. In a refinery process, petroleum is converted into petroleum intermediate prod- ucts, including gases, light/heavy naphtha, kerosene, diesel, light gas oil, heavy gas oil, and residue. From these intermediate refinery product streams, several fuels such as fuel gas, liquefied petroleum gas, gasoline, jet fuel, kerosene, auto diesel, and other heavy products such as lubricants, bunker oil, asphalt, and coke are obtained. In addition, these petroleum intermediates can be further processed and separated into products for petrochemical applications. In this chapter, petroleum will be introduced first. Petrochemicals will be intro- duced in the second part of the chapter. Petrochemicals – the main subject of this book – will address three major areas, (i) the production of the seven cornerstone petrochemicals: methane and synthesis gas, ethylene, propylene, butene, benzene, toluene, and xylenes; (ii) the uses of the seven cornerstone petrochemicals, and (iii) the technology to separate petrochemicals into individual components. 1.2 Petroleum Petroleum is derived from the Latin words “petra” and “oleum,” which means “rock” and “oil,” respectively. Petroleum also is known as crude oil or fossil fuel. It is a thick, flammable, yellow-to-black mixture of gaseous, liquid, and solid hydrocarbons formed from the remains of plants and animals. -
Molecular Dynamics Simulation Studies of Physico of Liquid
MD Simulation of Liquid Pentane Isomers Bull. Korean Chem. Soc. 1999, Vol. 20, No. 8 897 Molecular Dynamics Simulation Studies of Physico Chemical Properties of Liquid Pentane Isomers Seng Kue Lee and Song Hi Lee* Department of Chemistry, Kyungsung University, Pusan 608-736, Korea Received January 15, 1999 We have presented the thermodynamic, structural and dynamic properties of liquid pentane isomers - normal pentane, isopentane, and neopentane - using an expanded collapsed atomic model. The thermodynamic prop erties show that the intermolecular interactions become weaker as the molecular shape becomes more nearly spherical and the surface area decreases with branching. The structural properties are well predicted from the site-site radial, the average end-to-end distance, and the root-mean-squared radius of gyration distribution func tions. The dynamic properties are obtained from the time correlation functions - the mean square displacement (MSD), the velocity auto-correlation (VAC), the cosine (CAC), the stress (SAC), the pressure (PAC), and the heat flux auto-correlation (HFAC) functions - of liquid pentane isomers. Two self-diffusion coefficients of liq uid pentane isomers calculated from the MSD's via the Einstein equation and the VAC's via the Green-Kubo relation show the same trend but do not coincide with the branching effect on self-diffusion. The rotational re laxation time of liquid pentane isomers obtained from the CAC's decreases monotonously as branching increas es. Two kinds of viscosities of liquid pentane isomers calculated from the SAC and PAC functions via the Green-Kubo relation have the same trend compared with the experimental results. The thermal conductivity calculated from the HFAC increases as branching increases. -
Dehydrogenation by Heterogeneous Catalysts
Dehydrogenation by Heterogeneous Catalysts Daniel E. Resasco School of Chemical Engineering and Materials Science University of Oklahoma Encyclopedia of Catalysis January, 2000 1. INTRODUCTION Catalytic dehydrogenation of alkanes is an endothermic reaction, which occurs with an increase in the number of moles and can be represented by the expression Alkane ! Olefin + Hydrogen This reaction cannot be carried out thermally because it is highly unfavorable compared to the cracking of the hydrocarbon, since the C-C bond strength (about 246 kJ/mol) is much lower than that of the C-H bond (about 363 kJ/mol). However, in the presence of a suitable catalyst, dehydrogenation can be carried out with minimal C-C bond rupture. The strong C-H bond is a closed-shell σ orbital that can be activated by oxide or metal catalysts. Oxides can activate the C-H bond via hydrogen abstraction because they can form O-H bonds, which can have strengths comparable to that of the C- H bond. By contrast, metals cannot accomplish the hydrogen abstraction because the M- H bonds are much weaker than the C-H bond. However, the sum of the M-H and M-C bond strengths can exceed the C-H bond strength, making the process thermodynamically possible. In this case, the reaction is thought to proceed via a three centered transition state, which can be described as a metal atom inserting into the C-H bond. The C-H bond bridges across the metal atom until it breaks, followed by the formation of the corresponding M-H and M-C bonds.1 Therefore, dehydrogenation of alkanes can be carried out on oxides as well as on metal catalysts. -
Exposure Investigation Protocol: the Identification of Air Contaminants Around the Continental Aluminum Plant in New Hudson, Michigan Conducted by ATSDR and MDCH
Exposure Investigation Protocol - Continental Aluminum New Hudson, Lyon Township, Oakland County, Michigan Exposure Investigation Protocol: The Identification of Air Contaminants Around the Continental Aluminum Plant in New Hudson, Michigan Conducted by ATSDR and MDCH MDCH/ATSDR - 2004 Exposure Investigation Protocol - Continental Aluminum New Hudson, Lyon Township, Oakland County, Michigan TABLE OF CONTENTS OBJECTIVE/PURPOSE..................................................................................................... 3 RATIONALE...................................................................................................................... 4 BACKGROUND ................................................................................................................ 5 AGENCY ROLES .............................................................................................................. 6 ESTABLISHING CRITERIA ............................................................................................ 7 “Odor Events”................................................................................................................. 7 Comparison Values......................................................................................................... 7 METHODS ....................................................................................................................... 12 Instantaneous (“Grab”) Air Sampling........................................................................... 12 Continuous Air Monitoring.......................................................................................... -
Transition Metal Hydrides That Mediate Catalytic Hydrogen Atom Transfers
Transition Metal Hydrides that Mediate Catalytic Hydrogen Atom Transfers Deven P. Estes Submitted in partial fulfillment of the requirements for the degree of Doctor of Philosophy in the Graduate School of Arts and Sciences COLUMBIA UNIVERSITY 2014 © 2014 Deven P. Estes All Rights Reserved ABSTRACT Transition Metal Hydrides that Mediate Catalytic Hydrogen Atom Transfers Deven P. Estes Radical cyclizations are important reactions in organic chemistry. However, they are seldom used industrially due to their reliance on neurotoxic trialkyltin hydride. Many substitutes for tin hydrides have been developed but none have provided a general solution to the problem. Transition metal hydrides with weak M–H bonds can generate carbon centered radicals by hydrogen atom transfer (HAT) to olefins. This metal to olefin hydrogen atom transfer (MOHAT) reaction has been postulated as the initial step in many hydrogenation and hydroformylation reactions. The Norton group has shown MOHAT can mediate radical cyclizations of α,ω dienes to form five and six membered rings. The reaction can be done catalytically if 1) the product metalloradical reacts with hydrogen gas to reform the hydride and 2) the hydride can perform MOHAT reactions. The Norton group has shown that both CpCr(CO)3H and Co(dmgBF2)2(H2O)2 can catalyze radical cyclizations. However, both have significant draw backs. In an effort to improve the catalytic efficiency of these reactions we have studied several potential catalyst candidates to test their viability as radical cyclization catalysts. I investigate the hydride CpFe(CO)2H (FpH). FpH has been shown to transfer hydrogen atoms to dienes and styrenes. I measured the Fe–H bond dissociation free energy (BDFE) to be 63 kcal/mol (much higher than previously thought) and showed that this hydride is not a good candidate for catalytic radical cyclizations. -
Functionalized Aromatics Aligned with the Three Cartesian Axes: Extension of Centropolyindane Chemistry*
Pure Appl. Chem., Vol. 78, No. 4, pp. 749–775, 2006. doi:10.1351/pac200678040749 © 2006 IUPAC Functionalized aromatics aligned with the three Cartesian axes: Extension of centropolyindane chemistry* Dietmar Kuck‡ Fakultät für Chemie, Universität Bielefeld, Universitätsstraße 25, D-33615 Bielefeld, Germany Abstract: The unique geometrical features and structural potential of the centropolyindanes, a complete family of novel, 3D polycyclic aromatic hydrocarbons, are discussed with respect to the inherent orthogonality of their arene units. Thus, the largest member of the family, centrohexaindane, a topologically nonplanar hydrocarbon, is presented as a “Cartesian hexa- benzene”, because each of its six benzene units is stretched into one of the six directions of the Cartesian space. This feature is discussed on the basis of the X-ray crystal structures of centrohexaindane and two lower members of the centropolyindane family, viz. the parent tribenzotriquinacenes. Recent progress in multiple functionalization and extension of the in- dane wings of selected centropolyindanes is reported, including several highly efficient six- and eight-fold C–C cross-coupling reactions. Some particular centropolyindane derivatives are presented, such as the first twelve-fold functionalized centrohexaindane and a tribenzo- triquinacene bearing three mutually orthogonal phenanthroline groupings at its molecular pe- riphery. Challenges to further extend the arene peripheries of the tribenzotriquinacenes and fenestrindanes to give, eventually, graphite cuttings bearing a central bowl- or saddle-shaped center are outlined, as is the hypothetical generation of a “giant” nanocube consisting of eight covalently bound tribenzotriquinacene units. Along these lines, our recent discovery of a re- lated, solid-state supramolecular cube, containing eight molecules of a particular tri- bromotrinitrotribenzotriquinacene of the same absolute configuration, is presented for the first time. -
Energy and the Hydrogen Economy
Energy and the Hydrogen Economy Ulf Bossel Fuel Cell Consultant Morgenacherstrasse 2F CH-5452 Oberrohrdorf / Switzerland +41-56-496-7292 and Baldur Eliasson ABB Switzerland Ltd. Corporate Research CH-5405 Baden-Dättwil / Switzerland Abstract Between production and use any commercial product is subject to the following processes: packaging, transportation, storage and transfer. The same is true for hydrogen in a “Hydrogen Economy”. Hydrogen has to be packaged by compression or liquefaction, it has to be transported by surface vehicles or pipelines, it has to be stored and transferred. Generated by electrolysis or chemistry, the fuel gas has to go through theses market procedures before it can be used by the customer, even if it is produced locally at filling stations. As there are no environmental or energetic advantages in producing hydrogen from natural gas or other hydrocarbons, we do not consider this option, although hydrogen can be chemically synthesized at relative low cost. In the past, hydrogen production and hydrogen use have been addressed by many, assuming that hydrogen gas is just another gaseous energy carrier and that it can be handled much like natural gas in today’s energy economy. With this study we present an analysis of the energy required to operate a pure hydrogen economy. High-grade electricity from renewable or nuclear sources is needed not only to generate hydrogen, but also for all other essential steps of a hydrogen economy. But because of the molecular structure of hydrogen, a hydrogen infrastructure is much more energy-intensive than a natural gas economy. In this study, the energy consumed by each stage is related to the energy content (higher heating value HHV) of the delivered hydrogen itself. -
Thermal Conductivity Correlations for Minor Constituent Fluids in Natural
Fluid Phase Equilibria 227 (2005) 47–55 Thermal conductivity correlations for minor constituent fluids in natural gas: n-octane, n-nonane and n-decaneଝ M.L. Huber∗, R.A. Perkins Physical and Chemical Properties Division, National Institute of Standards and Technology, Boulder, CO 80305, USA Received 21 July 2004; received in revised form 29 October 2004; accepted 29 October 2004 Abstract Natural gas, although predominantly comprised of methane, often contains small amounts of heavier hydrocarbons that contribute to its thermodynamic and transport properties. In this manuscript, we review the current literature and present new correlations for the thermal conductivity of the pure fluids n-octane, n-nonane, and n-decane that are valid over a wide range of fluid states, from the dilute-gas to the dense liquid, and include an enhancement in the critical region. The new correlations represent the thermal conductivity to within the uncertainty of the best experimental data and will be useful for researchers working on thermal conductivity models for natural gas and other hydrocarbon mixtures. © 2004 Elsevier B.V. All rights reserved. Keywords: Alkanes; Decane; Natural gas constituents; Nonane; Octane; Thermal conductivity 1. Introduction 2. Thermal conductivity correlation Natural gas is a mixture of many components. Wide- We represent the thermal conductivity λ of a pure fluid as ranging correlations for the thermal conductivity of the lower a sum of three contributions: alkanes, such as methane, ethane, propane, butane and isobu- λ ρ, T = λ T + λ ρ, T + λ ρ, T tane, have already been developed and are available in the lit- ( ) 0( ) r( ) c( ) (1) erature [1–6]. -
Measurements of Higher Alkanes Using NO Chemical Ionization in PTR-Tof-MS
Atmos. Chem. Phys., 20, 14123–14138, 2020 https://doi.org/10.5194/acp-20-14123-2020 © Author(s) 2020. This work is distributed under the Creative Commons Attribution 4.0 License. Measurements of higher alkanes using NOC chemical ionization in PTR-ToF-MS: important contributions of higher alkanes to secondary organic aerosols in China Chaomin Wang1,2, Bin Yuan1,2, Caihong Wu1,2, Sihang Wang1,2, Jipeng Qi1,2, Baolin Wang3, Zelong Wang1,2, Weiwei Hu4, Wei Chen4, Chenshuo Ye5, Wenjie Wang5, Yele Sun6, Chen Wang3, Shan Huang1,2, Wei Song4, Xinming Wang4, Suxia Yang1,2, Shenyang Zhang1,2, Wanyun Xu7, Nan Ma1,2, Zhanyi Zhang1,2, Bin Jiang1,2, Hang Su8, Yafang Cheng8, Xuemei Wang1,2, and Min Shao1,2 1Institute for Environmental and Climate Research, Jinan University, 511443 Guangzhou, China 2Guangdong-Hongkong-Macau Joint Laboratory of Collaborative Innovation for Environmental Quality, 511443 Guangzhou, China 3School of Environmental Science and Engineering, Qilu University of Technology (Shandong Academy of Sciences), 250353 Jinan, China 4State Key Laboratory of Organic Geochemistry and Guangdong Key Laboratory of Environmental Protection and Resources Utilization, Guangzhou Institute of Geochemistry, Chinese Academy of Sciences, 510640 Guangzhou, China 5State Joint Key Laboratory of Environmental Simulation and Pollution Control, College of Environmental Sciences and Engineering, Peking University, 100871 Beijing, China 6State Key Laboratory of Atmospheric Boundary Physics and Atmospheric Chemistry, Institute of Atmospheric Physics, Chinese