Mechanism of Enhancement of Ferroelectricity of Croconic Acid with Temperature
Total Page:16
File Type:pdf, Size:1020Kb
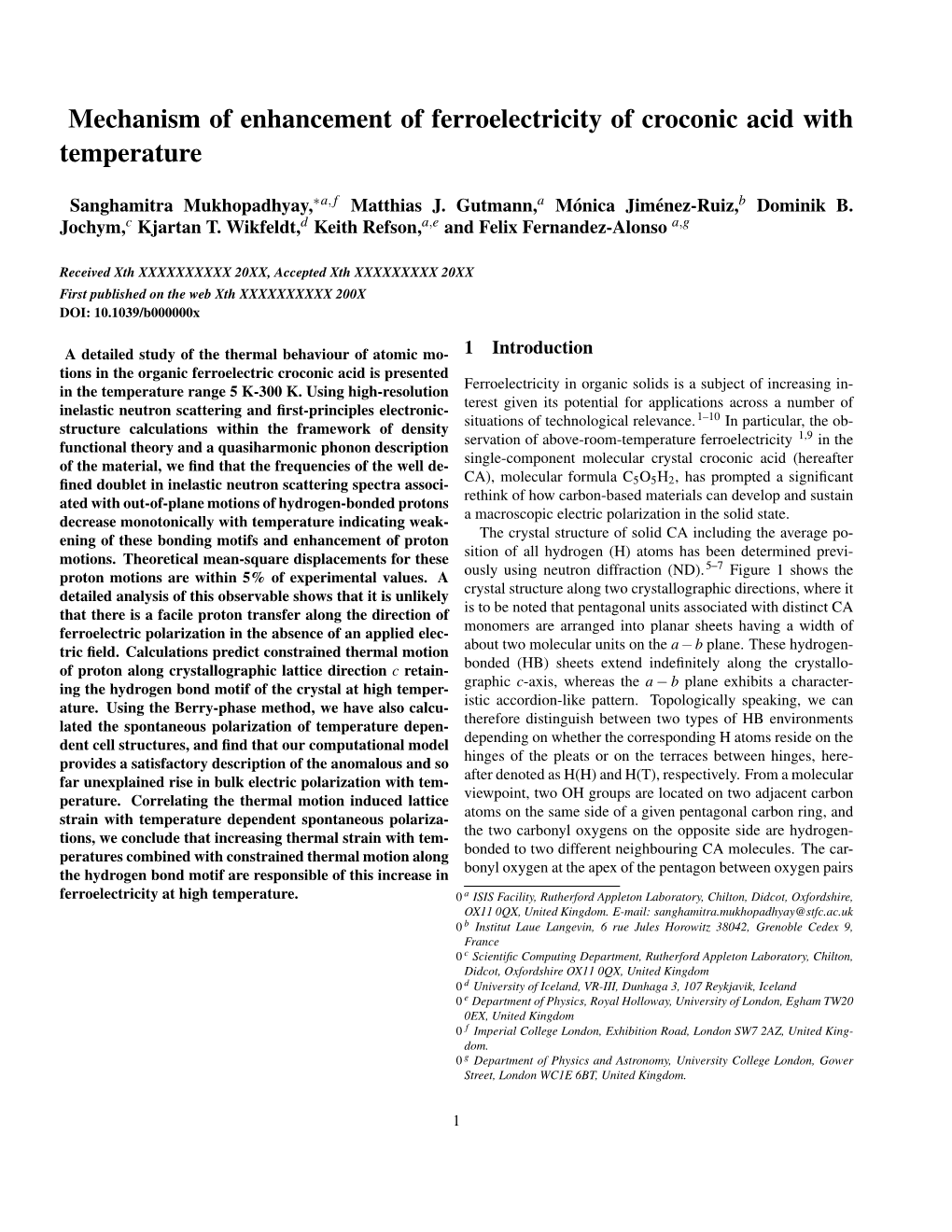
Load more
Recommended publications
-
Bond Donors for Selective Anion Recognition
FULL PAPER Deltamides and croconamides: expanding the range of dual H- bond donors for selective anion recognition Vincent E. Zwicker,[a],‡ Karen K. Y. Yuen,[a],‡ David G. Smith,[a] Junming Ho,[b] Lei Qin,[a] Peter Turner[a] and Katrina A. Jolliffe[a],* Abstract: Dual H-bond donors are widely used as recognition motifs match for Y-shaped anions such as carboxylates.3 Dual hydrogen in anion receptors. We report the synthesis of a library of dual H-bond bond donors have also been shown to have superior hydrogen receptors, incorporating the deltic and croconic acid derivatives, bond donicity to monodentate hydrogen bond donors of similar termed deltamides and croconamides, and a comparison of their acidity,4 providing improved anion binding capacity, together with anion binding affinities (for monovalent species) and Brønsted a decreased propensity for the receptor to be deprotonated by acidities to those of the well-established urea and squaramide dual H- basic anions. This latter property is of particular importance in the bond donor motifs. For dual H-bond cores with identical substituents, development of new anion binding motifs, since if a hydrogen the trend in Brønsted acidity is croconamides > squaramides bond donor is too acidic, it is no longer effective at binding to 5 >deltamides > ureas, with the croconamides found to be 10-15 pKa anions at neutral pH. Finding dual hydrogen bonding motifs with units more acidic than the corresponding ureas. In contrast to the the right balance between hydrogen bond donicity and Brønsted trends displayed by ureas, deltamides and squaramides, N,N’-dialkyl acidity should provide improved anion receptors and allow them croconamides displayed higher binding affinity to chloride than the to be tailored towards specific anions. -
Croconate Salts. New Bond-Delocalized Dianions, &Q
JOURNAL OF RESEARCH of the National Bureau of Standards Volume 85, No.2, March·April1980 Pseudo-Oxocarbons. Synthesis of 2, 1,3-Bis-, and 1, 2, 3-Tris (Dicyanomethylene) Croconate Salts. New Bond-Delocalized Dianions, "Croconate Violet" and "Croconate Blue"* Alexander J. Fatiadit National Bureau of Standards, Washington, D.C. 20234 October 24,1979 Synthesis and characteri zation of new bond·delocalized dianions, e.g., 2, 1,3·bis·, 1,2, 3·tris (di cyanomethyl. ene) croconate salts have been described. The dianions re ported represent a new class of aromati c, nonbenze· noid co mpounds, named pseudo·oxocarbons. A study of their physical, analytical and chemical properties offer a new direction in the chemistry of oxocarbons. Key words: Acid; aromatic; bond·delocalized; croco nic; diani on; malononitrile; nonbenzenoid; oxocarbon; salt; synthesis 1. Introduction molecular properties of the croconic salts (e.g. 2 , dipotas sium salt) were first seriously investigated when a symmetri The bright ye ll ow dipotassium croconate 1 and croconic cal, delocalized structure fo r the dianion 2 was proposed by acid (1 , K = H, 4,5-dihydroxy-4--cyclopentene-l,2,3-trione) Yamada et aJ. [3] in 1958. A few years later [4], the d i anion 2 were first isolated by Gmelin [1]' in 1825, from the black, ex· and the related deltate [5], squarate, rhodizonate, and plosive, side-reaction product (e.g. K6 C6 0 6 + KOC=COK), tetrahydroxyquinone anions were recognized by West et aJ. by the reaction of carbon with potassium hydroxide, in a [2,4] as members of a new class of aromatic oxocarbons pioneer, industrial attempt to manufacture potassium. -
Ion Batteries
i DEVELOPMENT OF NOVEL ASSEMBLY APPROACHES FOR SUPERIOR ELECTRODE MATERIALS OF LITHIUM‐ ION BATTERIES By Ruiming Huang A Dissertation submitted to the Graduate School-Newark Rutgers, the State University of New Jersey In partial fulfillment of the requirements For the degree of Doctor of Philosophy Graduate Program in Chemistry Written under the direction of Professor Huixin He And approved by ________________________ ________________________ ________________________ ________________________ Newark, New Jersey October, 2015 ii COPYRIGHT ©2015 Ruiming Huang ALL RIGHTS RESERVED ii ABSTRACT OF THE THESIS Development of Novel Assembly Approaches for Superior Electrode Materials of Lithium-Ion Batteries By Ruiming Huang Dissertation Director: Dr. Huixin He Rechargeable lithium ion batteries have attracted tremendous attention as “green” technology for electric vehicles and smart grids. In addition, the demand for flexible and high energy batteries has increased exponentially due to the growing need for smartphones and bio-devices over last few years. The conventional inorganic cathode materials (e.g., LiCoO2 and LiFePO4) for Lithium ion batteries are not flexible; they are also restricted by their low theoretical specific capacity. To satisfy the emerging large- scale applications of energy storage, new generation batteries should have high power and energy densities, and a long cycle life. In near term, new inorganic cathode and anode materials are developing to increase their capacity. In long term, the next generation batteries were proposed to be made from inexpensive renewable and/or recyclable resources via low energy consumption processes for energy sustainability with minimal environmental footprint. However, issues such as low electronic conductivity, large volume change during the charge/discharge cycles and dissolution of the active materials, commonly existed in these new electrode materials. -
Croconic Acid and Alkali Metal Croconate Salts: Some New Insights Into an Old Story
FULL PAPER Croconic Acid and Alkali Metal Croconate Salts: Some New Insights into an Old Story Dario Braga,*[a] Lucia Maini,[a] and Fabrizia Grepioni*[b] Abstract: The solid-state structures of a are described and compared with the interplanar separations lie in the narrow series of alkali metal salts of the cro- Li ,K and NH4 salts. Single crystals of range 3.12 ± 3.42 ä and do not necessa- 2À p conate dianion (C5O5 ) and of croconic croconic acid were obtained by crystal- rily reflect the presence of -stacking acid (H2C5O5) have been determined. lisation of croconic acid in the presence interactions. It is argued that the small The alkali metal croconates were ob- of HCl. Crystal structure determinations interplanar separation is the result of a 2À tained by ring contraction of rhodizonic showed that the C5O5 ions tend to compromise between packing of flat acid (H2C6O6), upon treatment with organize themselves in columns. The croconate units and the spherical cations alkali metal hydroxides and recrystalli- together with the water molecules that sation from water. The novel species fill the coordination spheres of the alkali Keywords: alkali metals ¥ Na C O ¥2H O, Rb C O and Cs C O , metal atoms. 2 5 5 2 2 5 5 2 5 5 croconates ¥ crystal engineering ¥ as well as the mixed hydrogencroconate/ oxocarbons ¥ stacking interactions croconate salt K3(HC5O5)(C5O5)¥2H2O Introduction supramolecular aggregation of the building blocks rather than from their nature. Crystal engineering, the bottom-up construction of crystalline Indeed most of the successful crystal-engineering experi- solids with desired arrangements of the component molecules ments have been conducted on simple systems, like guanidi- and ions,[1] has fuelled new interest in some old issues of nium and sulfonate ions,[5] organometallic acids,[6] halometal- structural chemistry. -
Anomalous Mechanical Behavior of the Deltic, Squaric and Croconic Cyclic Oxocarbon Acids
IOP Publishing Journal Title Journal XX (XXXX) XXXXXX https://doi.org/XXXX/XXXX Anomalous mechanical behavior of the deltic, squaric and croconic cyclic oxocarbon acids Francisco Colmenero1 1 Molecular Physics Department, Instituto de Estructura de la Materia (CSIC), Madrid, Spain E-mail: [email protected] Received xxxxxx Accepted for publication xxxxxx Published xxxxxx Abstract The structural and mechanical properties of the deltic, squaric and croconic cyclic oxocarbon acids were obtained using theoretical solid-state methods based in Density Functional Theory employing very demanding calculation parameters in order to yield realistic theoretical descriptions of these materials. The computed lattice parameters, bond distances, angles, and X-ray powder diffraction patterns of these materials were in excellent agreement with their experimental counterparts. The crystal structures of these materials were found to be mechanically stable since the calculated stiffness tensors satisfy the Born mechanical stability conditions. Furthermore, the values of the bulk modulus and their pressure derivatives, shear and Young moduli, Poisson ratio, ductility and hardness indices, as well as mechanical anisotropy measures of these materials were reported. A complete review of the literature concerning the negative Poisson ratio and negative linear compressibility phenomena is given together with the theoretical study of the mechanical behavior of cyclic oxocarbon acid materials. The deltic, squaric, and croconic acids in the solid state are highly anisotropic materials characterized by low hardness and relatively low bulk moduli. The three materials display small negative Poisson ratios. The croconic acid displays the phenomenon of negative linear compressibility for applied pressures larger than ~0.4 GPa directed along the direction of minimum Poisson ratio and undergoes a pressure induced phase transition at applied pressures larger than ~1.0 GPa. -
A Prebiotic Photochemical Synthesis of Glycerides
Proceedings of the 2nd WSEAS International Conference on BIOMEDICAL ELECTRONICS and BIOMEDICAL INFORMATICS A Prebiotic Photochemical Synthesis of Glycerides N. Aylward , Queensland University of Technology, Brisbane,Australia [email protected] Abstract: - The magnesium ion metalloporphyrin complex is shown to bind the ligands alkyne and carbon monoxide with migration and bridging between the metal ion and nitrogen bound ligands. The sequence of reactions to form the glyceride acyl bond is: 1. Up to four carbon monoxide molecules may be individually bound to the metal and migrate to the four vacant nitrogen sites to form bridged aziridine-2one rings. 2. The metal bound alkyne (e.g. propyne) reacts with a peripherally bound carbon monoxide to form a propanone ring compound. 3. The propanone ring ligand isomerises by a hydrogen shift to a 3-methyl butenone ligand with an activation energy of 0.112 h. 4. The 3-methyl butenone ligand forms an acyl bridge to a peripheral carbon monoxide with an activation energy of 0.007 h. 5. The excited state complex is greatly stabilised by protonation, whilst mild hydrogenation at the metal and positively charged carbon atom frees the alkene ester. 6. The activation energy to bond the three carbon monoxide entities bound to the peripheral nitrogen sites is 0.126 h. 7. Mild reduction at the peripheral nitrogen sites frees the mono-glyceride. The reactions have been shown to be feasible from the overall enthalpy changes in the ZKE approximation at the HF and MP2 /6-31G* level. Key-Words: - Prebiotic photochemical synthesis, mono-glycerides, carbon monoxide, propyne. -
Binder Comprising a Cyclic Oxocarbon
(19) TZZ¥__T (11) EP 3 184 497 A1 (12) EUROPEAN PATENT APPLICATION (43) Date of publication: (51) Int Cl.: 28.06.2017 Bulletin 2017/26 C03C 25/24 (2006.01) C03C 25/32 (2006.01) D04H 1/64 (2012.01) D04H 1/4209 (2012.01) (2006.01) (21) Application number: 15202578.9 C08G 16/00 (22) Date of filing: 23.12.2015 (84) Designated Contracting States: (72) Inventor: HJELMGAARD, Thomas AL AT BE BG CH CY CZ DE DK EE ES FI FR GB 3480 Fredensborg (DK) GR HR HU IE IS IT LI LT LU LV MC MK MT NL NO PL PT RO RS SE SI SK SM TR (74) Representative: Letzelter, Felix Phillip Designated Extension States: Meissner Bolte Patentanwälte BA ME Rechtsanwälte Partnerschaft mbB Designated Validation States: Widenmayerstraße 47 MA MD 80538 München (DE) (71) Applicant: Rockwool International A/S 2640 Hedehusene (DK) (54) BINDER COMPRISING A CYCLIC OXOCARBON (57) Described is an aqueous binder composition for selected from the group of ammonia, amines or any salts mineral fibers comprising thereof; a component (i) in the form of a cyclic oxocarbon; a component (iii) in the form of one or more carbohy- a component (ii) in the form of one or more compounds drates. EP 3 184 497 A1 Printed by Jouve, 75001 PARIS (FR) EP 3 184 497 A1 Description Field of the Invention 5 [0001] The present invention relates to an aqueous binder for mineral fibre products, a method of producing a bonded mineral fibre product using said binder, and a mineral fibre product comprising mineral fibres in contact with a cured binder. -
Synthesis of Superelectrophiles from Small Oxocarbon Compounds
Dissertation zur Erlangung des Doktorgrades der Fakultät für Chemie und Pharmazie der Ludwig-Maximilians-Universität München Synthesis of Superelectrophiles from Small Oxocarbon Compounds Manuel Tobias Schickinger aus Gräfelfing 2018 Erklärung Diese Dissertation wurde im Sinne von § 7 der Promotionsordnung vom 28. November 2011 von Herrn Prof. Dr. Andreas J. Kornath betreut. Eidesstattliche Versicherung Diese Dissertation wurde selbständig, ohne unerlaubte Hilfe erarbeitet. München, den 3. Januar 2019 ............................................................................. Manuel Schickinger Dissertation eingereicht am 15. November 2018 1. Gutachter: Prof. Dr. Andreas Kornath 2. Gutachter: Prof. Dr. Thomas Klapötke Mündliche Prüfung am 13. Dezember 2018 „Mir koe die Wege, auf dee die Meshe zur Erketis gelage, fast ebenso euderugsürdig or ie die Natur der Dige selst.“ Johannes Kepler Danksagung Zuerst möchte ich mich besonders bei Herrn Prof. Dr. Andreas J. Kornath für die freundliche Aufnahme in seinem Arbeitskreis, für das sehr interessante und herausfordernde Thema dieser Dissertation, sein Vertrauen und seine Unterstützung sowie die Freiheit, in wissenschaftlicher Hinsicht meine Ideen zu verwirklichen, bedanken. Auch für die Möglichkeit, an zahlreichen Konferenzen teilnehmen zu dürfen, möchte ich mich ganz herzlich bedanken. Des Weiteren möchte ich Herrn Prof. Dr. Thomas M. Klapötke für die Übernahme der Zweitkorrektur danken. Als nächstes gilt mein Dank Joe und Can, die mich so freundlich in diesem Arbeitskreis aufgenommen haben und mir als Mentoren die nicht ganz alltäglichen Arbeitstechniken beigebracht haben. Darüber hinaus sind sie auch noch auf privater Ebene zu guten Freunden geworden. Domi, Ines, Flo, Yvonne, Michi, Chris, Steffi, Alan, Lukas, Julia, Nedz und Marie möchte ich für die einzigartige Arbeitsatmosphäre danken. Es war jeden Tag eine Freude, mit euch zusammenzuarbeiten und in zahlreichen konstruktiven Gesprächen chemische Probleme zu diskutieren. -
Lit Seminar T1
Lead-Free Ferroelectric Materials: BaTiO3 and Beyond By Claire McGhee Since its discovery in the 1880’s the field piezoelectric materials has grown and the materials have been incorporated into many devices in modern technology including actuators, transducers, generators, sensors, capacitors, and oscillators.1 Piezoelectric materials gain their unique electromechanical properties because of their non-centrosymmetric crystal structure.2 This structure affords two distinct groups of materials, those which have an induced- dipole and those with a permanent dipole. Materials with a permanent dipole tend to have a stronger piezoelectric response, and are referred to as ferroelectric materials. Because of the strong piezoelectric properties ferroelectric materials exhibit, they have been well developed in the field of materials science. Of these materials, the most studied and applied materials are perovskite structure polycrystalline ceramics.3 Recent advancements in the study of perovskite thin films is continuing to further application of these classic materials. To a lesser degree, semicrystalline polymers and small-molecule single-crystals have been developed. In the past, both polymer and small-molecule ferroelectric materials tended to have a smaller piezo-response, a lower Curie temperature, and a smaller spontaneous polarization, however, recently, single- crystal small-molecule ferroelectric materials have been characterized which have properties comparable with perovskite ceramics. This talk will review one of the new applications of perovskite ultrathin films and the properties of these newly developed ferroelectric materials. One unique aspect of ferroelectric materials is the permanent dipole within the material, the orientation of which is measured by the sign of its spontaneous polarization. When an electric field is applied to a ferroelectric material, its spontaneous polarization can be reoriented, or flipped. -
Squaric Acid Adsorption and Oxidation at Gold and Platinum Electrodes
Accepted Manuscript Squaric acid adsorption and oxidation at gold and platinum electrodes William Cheuquepán, Jorge Martínez-Olivares, Antonio Rodes, José Manuel Orts PII: S1572-6657(17)30725-7 DOI: doi:10.1016/j.jelechem.2017.10.023 Reference: JEAC 3583 To appear in: Journal of Electroanalytical Chemistry Received date: 28 July 2017 Revised date: 6 October 2017 Accepted date: 10 October 2017 Please cite this article as: William Cheuquepán, Jorge Martínez-Olivares, Antonio Rodes, José Manuel Orts , Squaric acid adsorption and oxidation at gold and platinum electrodes. The address for the corresponding author was captured as affiliation for all authors. Please check if appropriate. Jeac(2017), doi:10.1016/j.jelechem.2017.10.023 This is a PDF file of an unedited manuscript that has been accepted for publication. As a service to our customers we are providing this early version of the manuscript. The manuscript will undergo copyediting, typesetting, and review of the resulting proof before it is published in its final form. Please note that during the production process errors may be discovered which could affect the content, and all legal disclaimers that apply to the journal pertain. ACCEPTED MANUSCRIPT Squaric acid adsorption and oxidation at gold and platinum electrodes William Cheuquepánb, Jorge Martínez-Olivares, Antonio Rodesa,b,*, José Manuel Ortsa,b a Departamento de Química Física and b Instituto Universitario de Electroquímica Universidad de Alicante Apartado 99, E-03080 Alicante, Spain *Corresponding author E-mail: [email protected] Phone and fax: 965909814 Abstract The adsorption and oxidation of squaric acid (3,4-dihydroxycyclobut-3-ene-1,2-dione, H2C4O4, SQA) at platinum and gold electrodes were studied spectroelectrochemically in perchloric acid solutions. -
2D Cocrystallization from H‑Bonded Organic Ferroelectrics Donna A
University of Nebraska - Lincoln DigitalCommons@University of Nebraska - Lincoln Stephen Ducharme Publications Research Papers in Physics and Astronomy 1-2016 2D Cocrystallization from H‑Bonded Organic Ferroelectrics Donna A. Kunkel Univeristy of Nebraska-Lincoln, [email protected] James Hooper Jagiellonian University, [email protected] Benjamin Bradley University of Nebraska-Lincoln Lisa Schlueter California State University at San Bernadino Tom Rasmussen University of Nebraska-Lincoln See next page for additional authors Follow this and additional works at: http://digitalcommons.unl.edu/physicsducharme Part of the Atomic, Molecular and Optical Physics Commons, and the Condensed Matter Physics Commons Kunkel, Donna A.; Hooper, James; Bradley, Benjamin; Schlueter, Lisa; Rasmussen, Tom; Costa, Paulo; Beniwal, Sunit; Ducharme, Stephen; Zurek, Eva; and Enders, Axel, "2D Cocrystallization from H‑Bonded Organic Ferroelectrics" (2016). Stephen Ducharme Publications. 106. http://digitalcommons.unl.edu/physicsducharme/106 This Article is brought to you for free and open access by the Research Papers in Physics and Astronomy at DigitalCommons@University of Nebraska - Lincoln. It has been accepted for inclusion in Stephen Ducharme Publications by an authorized administrator of DigitalCommons@University of Nebraska - Lincoln. Authors Donna A. Kunkel, James Hooper, Benjamin Bradley, Lisa Schlueter, Tom Rasmussen, Paulo Costa, Sunit Beniwal, Stephen Ducharme, Eva Zurek, and Axel Enders This article is available at DigitalCommons@University of Nebraska - Lincoln: http://digitalcommons.unl.edu/physicsducharme/106 Published in Journal of Physical Chemistry Letters 7:3 (January 2016), pp. 435−440; doi: 10.1021/acs.jpclett.5b02472 Copyright © 2016 American Chemical Society. Used by permission. Submitted November 5, 2015; accepted January 11, 2016; published January 11, 2016. -
Based Framework
J. Chem. Sci. Vol. 127, No. 2, February 2015, pp. 257–264. c Indian Academy of Sciences. DOI 10.1007/s12039-015-0771-8 Exploration of the structural features and magnetic behaviour in a novel 3-dimensional interpenetrating Co(II)-based framework SOUMYABRATA GOSWAMI, AMIT KUMAR MONDAL and SANJIT KONAR∗ Department of Chemistry, IISER Bhopal, Indore By-pass Road, Bhauri, Bhopal 462 066, India e-mail: [email protected] MS received 29 September 2014; accepted 9 October 2014 Abstract. A new Co(II)-based three-dimensional (3D) framework having the molecular formula [Co(C4O4) (4-bpmh))H2O)2]n· 2nH2O·2nMeOH·(1) (4-bpmh = N, N-bis-pyridin-4-ylmethylene-hydrazine) has been syn- thesized using a mixed ligand system and characterized by elemental analysis, IR spectroscopy, thermogravi- metric analysis, single crystal X-ray diffraction and variable temperature magnetic study. The framework is 2− constructed by the bridging squarate (C4O4 ) and N, N-bis-pyridin-4-ylmethylene-hydrazine (4-bpmh) ligands and interpenetration of the 2D grid-like frameworks at definite angles gives rise to 2D →3D inclined polycate- nation with sql/Shubnikov tetragonal plane net topology. Extensive non-covalent interactions (H-bonding as well as π ···π interactions) are also observed which stabilises the 3D arrangement. Additionally, complex 1 contains 1D channels of large dimensions (10.91 × 11.78 Å2) that runs along the b-axis. Variable temperature 4 DC magnetic susceptibility study reveals dominant spin–orbit coupling effect typical of the T1g ground state of octahedral high-spin Co(II) ion at a higher temperature range. Keywords.