Deriving Asteroid Mineralogies from Reflectance Spectra
Total Page:16
File Type:pdf, Size:1020Kb
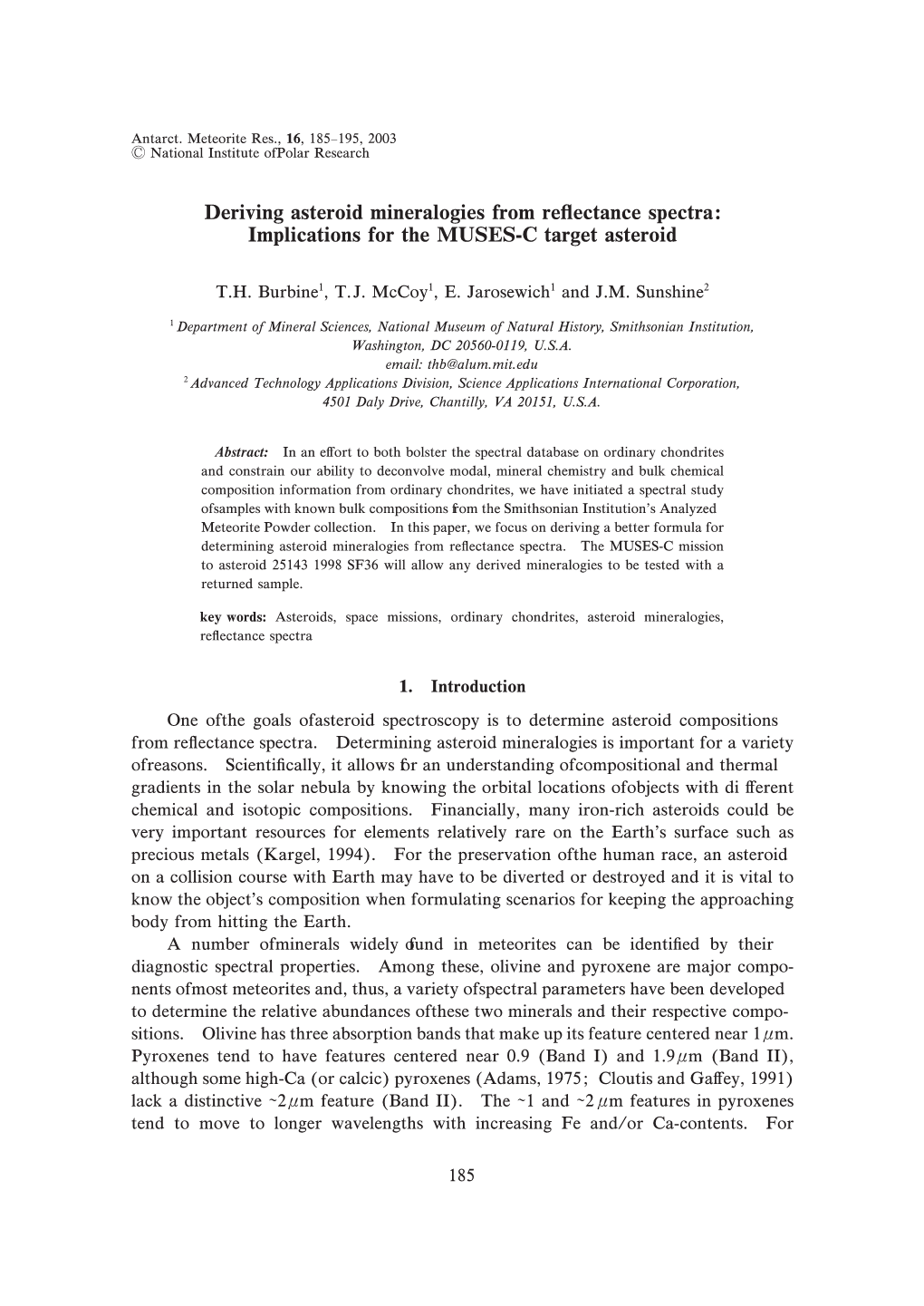
Load more
Recommended publications
-
Meteorite Collections: Sample List
Meteorite Collections: Sample List Institute of Meteoritics Department of Earth and Planetary Sciences University of New Mexico October 01, 2021 Institute of Meteoritics Meteorite Collection The IOM meteorite collection includes samples from approximately 600 different meteorites, representative of most meteorite types. The last printed copy of the collection's Catalog was published in 1990. We will no longer publish a printed catalog, but instead have produced this web-based Online Catalog, which presents the current catalog in searchable and downloadable forms. The database will be updated periodically. The date on the front page of this version of the catalog is the date that it was downloaded from the worldwide web. The catalog website is: Although we have made every effort to avoid inaccuracies, the database may still contain errors. Please contact the collection's Curator, Dr. Rhian Jones, ([email protected]) if you have any questions or comments. Cover photos: Top left: Thin section photomicrograph of the martian shergottite, Zagami (crossed nicols). Brightly colored crystals are pyroxene; black material is maskelynite (a form of plagioclase feldspar that has been rendered amorphous by high shock pressures). Photo is 1.5 mm across. (Photo by R. Jones.) Top right: The Pasamonte, New Mexico, eucrite (basalt). This individual stone is covered with shiny black fusion crust that formed as the stone fell through the earth's atmosphere. Photo is 8 cm across. (Photo by K. Nicols.) Bottom left: The Dora, New Mexico, pallasite. Orange crystals of olivine are set in a matrix of iron, nickel metal. Photo is 10 cm across. (Photo by K. -
Breve Histórico Dos Meteoritos Brasileiros
Parte 1 Breve histórico dos meteoritos brasileiros Maria Elizabeth Zucolotto (MN/UFRJ) Os meteoritos se prestam ao estudo das condições e processos físicos da formação do sistema solar. São fragmentos de corpos em diversos estágios de diferenciação planetária, sendo encontrados desde meteoritos primitivos, de composição solar, até representantes da crosta, manto e núcleo de corpos planetários diferenciados. A história dos meteoritos brasileiros está diretamente ligada à história da meteorítica, pois o Bendegó foi descoberto em 1784 quando ainda se desconhecia a natureza extraterrestre dos meteoritos. O Bendegó foi durante muitos anos o maior meteorito em exposição em um museu. O Brasil possui hoje apenas 62 meteoritos certificados, alguns muito importantes como o Angra dos Reis, que deu origem a uma classe de meteoritos, os “angritos”. Pedras sagradas Embora os meteoritos só tenham sido aceitos pela ciência como objetos de origem extraterrestre no início do século 19, o fenômeno de queda de rochas e ferro sobre a Terra (meteoros e bólidos) era conhecido desde a antiguidade. Papiros egípcios, de 4 mil anos, registram objetos luminosos riscando os céus numa representação típica de queda de meteoritos, isto é, queda de objetos sólidos no chão. Escritos gregos, de 3,5 mil e 2,5 mil anos, mencionam a queda de pedras e ferro do céu. Provavelmente pela natureza extraterrestre e supostos poderes mágicos, al- guns meteoritos foram objetos de veneração em várias civilizações, dos quais só restaram algumas descrições históricas. A mais interessante é a de Tito Lívio rela- tando que, em 204 AEC, a pedra negra que simbolizava a Magna Mater (Grande Mãe, também chamada Cibele), foi levada para Roma em situação interessante: os exércitos de Aníbal tinham penetrado nos territórios romanos disseminan- do o pânico entre a população. -
Bartoschewitz - Catalogue of Meteorites
BARTOSCHEWITZ - CATALOGUE OF METEORITES *FALL TOTAL BC- BC - NAME COUNTRY FIND WEIGHT TYPE No. SPECIMEN WEIGHT (kg) (gms) 1.1 CHONDRITES - ORDINARY OLIVINE BRONZITE CHONDRITES ACFER 005 Algeria March 1989 0,115 H 3.9/4 613.1 cut endpiece 32,70 ACFER 006 Algeria March 1989 0,561 H 3.9/4 614.1 slice 1,30 ACFER 011 Algeria 1989 3,8 H 5 399.1 cut fragm. 3,00 ACFER 020 Algeria 1989 0,708 H 5 401.1 cut fragm. 2,50 ACFER 028 Algeria 1989 3,13 H 3.8 844.1 part slice 1,70 ACFER 050 Algeria 1989 1,394 H 5-6 443.1 complete slice 105,00 ACFER 084 Algeria Apr. 16, 1990 6,3 H 5 618.1 cut corner piece 12,60 ACFER 089 Algeria 1990 0,682 H 5 437.1 complete slice 62,00 ACFER 098 Algeria 1990 5,5 H 5 615.1 cut fragment 29,20 ACFER 222 Algeria 1991 0,334 H 5-6 536.1 cut fragm. with crust 2,50 ACFER 284 Algeria 1991 0,12 H 5 474.1 slice 11,00 ACHILLES USA, Kansas 1924 recogn. 1950 16 H 5 314.1 slice 3,40 ACME USA, New Mexico 1947 75 H 5 303.1 slice 10,80 AGEN France *Sept. 5, 1815 30 H 5 208.1 fragm. with crust 54,40 ALAMOGORDO USA, New Mexico 1938 13,6 H 5 2.1 fragment 0,80 ALLEGAN USA *July 10, 1899 35 H 5 276.0 5 small fragments 1,52 ALLEGAN USA *July 10, 1899 35 H 5 276.1 5 small fragments 1,50 ALLEGAN USA *July 10, 1899 35 H 5 276.2 chondrules 0,02 ALLEN USA, Texas 1923 recogn. -
Meteorite Collections: Catalog
Meteorite Collections: Catalog Institute of Meteoritics Department of Earth and Planetary Sciences University of New Mexico July 25, 2011 Institute of Meteoritics Meteorite Collection The IOM meteorite collection includes samples from approximately 600 different meteorites, representative of most meteorite types. The last printed copy of the collection's Catalog was published in 1990. We will no longer publish a printed catalog, but instead have produced this web-based Online Catalog, which presents the current catalog in searchable and downloadable forms. The database will be updated periodically. The date on the front page of this version of the catalog is the date that it was downloaded from the worldwide web. The catalog website is: Although we have made every effort to avoid inaccuracies, the database may still contain errors. Please contact the collection's Curator, Dr. Rhian Jones, ([email protected]) if you have any questions or comments. Cover photos: Top left: Thin section photomicrograph of the martian shergottite, Zagami (crossed nicols). Brightly colored crystals are pyroxene; black material is maskelynite (a form of plagioclase feldspar that has been rendered amorphous by high shock pressures). Photo is 1.5 mm across. (Photo by R. Jones.) Top right: The Pasamonte, New Mexico, eucrite (basalt). This individual stone is covered with shiny black fusion crust that formed as the stone fell through the earth's atmosphere. Photo is 8 cm across. (Photo by K. Nicols.) Bottom left: The Dora, New Mexico, pallasite. Orange crystals of olivine are set in a matrix of iron, nickel metal. Photo is 10 cm across. (Photo by K. -
CONTENTS – W Through Z
67th Annual Meteoritical Society Meeting (2004) alpha_w-z.pdf CONTENTS – W through Z The Location of Martian Atmospheric Argon in Three Martian Basalts: Controls Exerted by Shock Effects E. L. Walton, S. P. Kelley, and J. G. Spray ............................................................................................ 5182 Core Formation in Planetesimals: Constraints from Siderophile Elements in Ureilites P. H. Warren .......................................................................................................................................... 5223 Lunar Meteorite Yamato-983885: A Relatively KREEPy Regolith Breccia Not Paired with Y-791197 P. H. Warren and J. C. Bridges.............................................................................................................. 5095 Extensive Melting of Amoeboid-Olivine Inclusions J. T. Wasson, A. E. Rubin, and J. M. Trigo-Rodriguez........................................................................... 5202 Fabric Analysis of Allende Matrix Using EBSD L. E. Watt, P. A. Bland, S. S. Russell, and D. J. Prior............................................................................ 5037 Unraveling the Exposure Histories of Aubrites K. C. Welten, K. Nishiizumi, D. J. Hillegonds, M. W. Caffee, and J. Masarik....................................... 5220 Nitrogen-Mapping and Nitrogen-XANES Spectroscopy of Interplanetary Dust Particles S. Wirick, G. J. Flynn, L. P. Keller, and C. Jacobsen ............................................................................ 5092 Further -
STUDIES of Brazillan METEORITES. II. the AVANHANDAVA CHONDRITE: MINERALOGY, PETROLOGY and CHEMISTRY
Revista Brasileira de Geocüncias Vclume B, 1976 201 STUDIES OF BRAZILlAN METEORITES. II. THE AVANHANDAVA CHONDRITE: MINERALOGY, PETROLOGY AND CHEMISTRY W. PAAR", K. KEIL, C.B. GOMES"", Department of Geology and Institute of Meteoritics, University of New Mexico,Albuquerque, New México 87131, USA and E. JAROSEWICH Department of Mineral Sciences, Smithsonian Institution, National Museum of Natural History, Washington, D.e. 20560, USA ABSTRACT A description of the Avanhandava, State of São Paulc. Braail; meteorite, which fell in 1952, is given using optical, microprobe, and bulk chemical data, Thia Hcgroup- or olivine -bronzite chondrite contains olivine (Fa I7.3), bronzite (Fs 16'5)' díopeide (Wo45.3En49'O Fs5.7), oli goclase (Or 5.4 Ab 84.1 An IO' 4)' whitlockite, chlorapatite, kamacite, taenite (plessite), -troilite, chromite, native copper, pentlandite, ilmenite, bravoite, mackinawite, and chalcopyrrhotite. On the basis of mineral and bulk compositions as well as its texture, the Avanhandava meteorite is classified as an H4 chondrite. RESU MO Dados ópticos e .. químicos são fornecidos para o meteorito de Avanhandáva, Estado de São Paulo, Brasil, cuja queda teve lugar em 1952. Este olivina-bronzita condrito, pertencente ,,=0 grupo H, consiste em, olivina (FaI7,3)' bronzita (Fs I6,5)' diopsídio (Wo45,3 En49,o FS5,7)' oligoclásio (Or 5,4 Ab 84, 1 An 10,4)' whitlockita, cloroapatita, kamacita, taenita [plesaita], troilita, crornitá, cobre nativo, pentlandita, ilmenita, bravoita, mackinawita e calcopirita. Com base em dados químicos (composição mineral e global] e' texturais, o meteorito de Avanhandava é classificado como um con drito H4. INTRODUCTION The Avanhandava, State of São Paulo, Brazil, stone meteorite fell . -
Catalogue of Meteorites from South America Springerbriefs in Earth System Sciences
SPRINGER BRIEFS IN EARTH SYSTEM SCIENCES SOUTH AMERICA AND THE SOUTHERN HEMISPHERE Rogelio Daniel Acevedo Maximiliano C. L. Rocca Víctor Manuel García Catalogue of Meteorites from South America SpringerBriefs in Earth System Sciences South America and the Southern Hemisphere Series editors Gerrit Lohmann Jorge Rabassa Justus Notholt Lawrence A. Mysak Vikram Unnithan For further volumes: http://www.springer.com/series/10032 Rogelio Daniel Acevedo Maximiliano C. L. Rocca Víctor Manuel García Catalogue of Meteorites from South America 123 Rogelio Daniel Acevedo Víctor Manuel García Centro Austral de Investigaciones Centro Austral de Investigaciones Científicas Científicas Tierra del Fuego Tierra del Fuego Argentina Argentina Maximiliano C. L. Rocca The Planetary Society Buenos Aires Argentina There may be instances where the authors have been unable to trace or contact the copyright holders. If notified the publisher will be pleased to rectify any errors or omissions at the earliest opportunity. ISSN 2191-589X ISSN 2191-5903 (electronic) ISBN 978-3-319-01924-6 ISBN 978-3-319-01925-3 (eBook) DOI 10.1007/978-3-319-01925-3 Springer Cham Heidelberg New York Dordrecht London Library of Congress Control Number: 2013949406 Ó The Author(s) 2014 This work is subject to copyright. All rights are reserved by the Publisher, whether the whole or part of the material is concerned, specifically the rights of translation, reprinting, reuse of illustrations, recitation, broadcasting, reproduction on microfilms or in any other physical way, and transmission or information storage and retrieval, electronic adaptation, computer software, or by similar or dissimilar methodology now known or hereafter developed. Exempted from this legal reservation are brief excerpts in connection with reviews or scholarly analysis or material supplied specifically for the purpose of being entered and executed on a computer system, for exclusive use by the purchaser of the work. -
Thirty-Eighth Lunar and Planetary Science Conference Program Of
38th LUNAR AND PLANETARY SCIENCE CONFERENCE PROGRAM OF TECHNICAL SESSIONS SPONSORED BY LUNAR AND PLANETARY INSTITUTE NASA JOHNSON SPACE CENTER LPI THIRTY-EIGHTH LUNAR AND PLANETARY SCIENCE CONFERENCE Program of Technical Sessions March 12–16, 2007 Sponsored by Lunar and Planetary Institute NASA Johnson Space Center Program Committee Stephen Mackwell, Co-Chair, Lunar and Planetary Institute Eileen Stansbery, Co-Chair, NASA Johnson Space Center Robert Anderson, Jet Propulsion Laboratory Nancy Chabot, Johns Hopkins University Catherine Corrigan, Johns Hopkins University David Draper, University of New Mexico Herbert Frey, NASA Goddard Space Flight Center Yulia Goreva, University of Arizona Tracy Gregg, University at Buffalo Terry Hurford, NASA Goddard Space Flight Center Ross Irwin, Smithsonian Institution Randy Korotev, Washington University at St. Louis Don Korycansky, University of California Santa Cruz Monika Kress, San Jose State University Rachel Lentz, University of Hawaii Karl Mitchell, Jet Propulsion Laboratory Daniel Nunes, Lunar and Planetary Institute Elisabetta Pierazzo, University of Arizona Louise Prockter, Johns Hopkins University Frans Rietmeijer, University of New Mexico Paul Schenk, Lunar and Planetary Institute Stephanie Shipp, Lunar and Planetary Institute Suzanne Smrekar, Jet Propulsion Laboratory David Vaniman, Los Alamos National Laboratory Michael Weisberg, Kingsborough College and the University of New York David Williams, Arizona State University James Zimbelman, Smithsonian Institution Michael Zolensky, NASA Johnson Space Center GUIDE TO TECHNICAL SESSIONS AND ACTIVITIES Sunday Evening, 5:00 p.m. LPI Hess Room Registration LPI Great Room Reception LPI Berkner Rooms Open House Education and Public Outreach Displays: p. 1 Accessing the Solar System Through Educational Products Monday Morning, 8:30 a.m. Crystal Ballroom A Mars Polar and Glacial Processes p. -
Estudo Gravimétrico Da Região Do Cone Do
ESTUDO PETROGRÁFICO, METALOGRÁFICO E GEOQUÍMICO DOS METEORITOS BOCAIUVA E JOÃO PINHEIRO – MINAS GERAIS i ii FUNDAÇÃO UNIVERSIDADE FEDERAL DE OURO PRETO Reitor João Luiz Martins Vice-Reitor Antenor Rodrigues Barbosa Júnior Pró-Reitor de Pesquisa e Pós-Graduação Tanus Jorge Nagem ESCOLA DE MINAS Diretor José Geraldo Arantes de Azevedo Brito Vice-Diretor Wilson Trigueiro de Souza DEPARTAMENTO DE GEOLOGIA Chefe Issamu Endo iii EVOLUÇÃO CRUSTAL E RECURSOS NATURAIS iv CONTRIBUIÇÕES ÀS CIÊNCIAS DA TERRA – SÉRIE M VOL. 67 DISSERTAÇÃO DE MESTRADO N° 289 ESTUDO PETROGRÁFICO, METALOGRÁFICO E GEOQUÍMICO DOS METEORITOS BOCAIUVA E JOÃO PINHEIRO MINAS GERAIS Flavia Noelia Pucheta Orientador Antonio Luciano Gandini Co-orientadores Flavio Sandro Lays Cassino Maria Elizabeth Zucolotto Dissertação apresentada ao Programa de Pós-Graduação em Evolução Crustal e Recursos Naturais do Departamento de Geologia da Escola de Minas da Universidade Federal de Ouro Preto como requisito parcial à obtenção do Título de Mestre em Ciências Naturais, Área de Concentração: Petrogênese/Depósitos Minerais/Gemologia OURO PRETO 2010 v Universidade Federal de Ouro Preto – http://www.ufop.br Escola de Minas - http://www.em.ufop.br Departamento de Geologia - http://www.degeo.ufop.br/ Programa de Pós-Graduação em Evolução Crustal e Recursos Naturais Campus Morro do Cruzeiro s/n - Bauxita 35.400-000 Ouro Preto, Minas Gerais Tel. (31) 3559-1600, Fax: (31) 3559-1606 e-mail: [email protected] Os direitos de tradução e reprodução reservados. Nenhuma parte desta publicação poderá ser gravada, armazenada em sistemas eletrônicos, fotocopiada ou reproduzida por meios mecânicos ou eletrônicos ou utilizada sem a observância das normas de direito autoral. -
1 Thermal and Impact History of the H Chondrite Parent Asteroid
Thermal and Impact History of the H Chondrite Parent Asteroid during Metamorphism: Constraints from Metallic Fe-Ni Edward R. D. Scott1*, Tatiana V. Krot1, Joseph I. Goldstein2, and Shigeru Wakita1,3 1Hawai‘i Institute of Geophysics and Planetology, University of Hawai‘i at Mānoa, Honolulu, HI, 96822, USA 2Dept. of Mechanical and Industrial Engineering, University of Massachusetts, Amherst, MA 01003, USA 3Center for Computational Astrophysics, National Astronomical Observatory of Japan, 2-21-1 Osawa, Mitaka, Tokyo 181-8588, Japan Submitted to GCA July 29, 2013 * Corresponding author. Email address: [email protected] (E. Scott) Revised: November 26, 2013 Revised again: March 7, 2014. Accepted March 26. Abstract: We have studied cloudy taenite, metallographic cooling rates, and shock effects in 30 H3-6 chondrites to elucidate the thermal and early impact history of the H chondrite parent body. We focused on H chondrites with old Ar-Ar ages (>4.4 Gyr) and unshocked and mildly shocked H chondrites, as strongly shocked chondrites with such old ages are very rare. Cooling rates for most H chondrites at 500 °C are 10-50 °C/Myr and do not decrease systematically with increasing petrologic type as predicted by the onion-shell model in which types 3 to 5 are arranged in concentric layers around a type 6 core. Some type 4 chondrites cooled slower than some type 6 chondrites and type 3 chondrites did not cool faster than other types, contrary to the onion-shell model. Cloudy taenite particle sizes, which range from 40 to 120 nm, are inversely correlated with metallographic cooling rates and show that the latter were not compromised by shock heating. -
The Accretion and Impact History of the Ordinary Chondrite Parent Bodies
Available online at www.sciencedirect.com ScienceDirect Geochimica et Cosmochimica Acta 200 (2017) 201–217 www.elsevier.com/locate/gca The accretion and impact history of the ordinary chondrite parent bodies Terrence Blackburn a,⇑, Conel M.O’D. Alexander b, Richard Carlson b, Linda T. Elkins-Tanton c a Earth and Planetary Sciences, University of California, Santa Cruz, Santa Cruz, CA 95064, United States b Department of Terrestrial Magnetism, Carnegie Institution for Science, Washington, DC 20015, United States c School of Earth and Space Exploration, Arizona State University, Tempe, AZ 85287, United States Received 12 February 2016; accepted in revised form 24 November 2016; Available online 9 December 2016 Abstract A working timeline for the history of ordinary chondrites includes chondrule formation as early as 0–2 Ma after our Solar System’s earliest forming solids (CAIs), followed by rapid accretion into undifferentiated planetesimals that were heated inter- nally by 26Al decay and cooled over a period of tens of millions of years. There remains conflict, however, between metallo- graphic cooling rate (Ni-metal) and radioisotopic thermochronometric data over the sizes and lifetimes of the chondrite parent bodies, as well as the timing of impact related disruptions. The importance of establishing the timing of parent body disruption is heightened by the use of meteorites as recorders of asteroid belt wide disruption events and their use to interpret Solar System dynamical models. Here we attempt to resolve these records by contributing new 207Pb–206Pb data obtained on phosphates isolated from nine previously unstudied ordinary chondrites. These new results, along with previously published Pb-phosphate, Ni-metal and thermometry data, are interpreted with a series of numerical models designed to simulate the thermal evolution for a chondrite parent body that either remains intact or is disrupted by impact prior to forming smaller unsorted ‘‘rubble piles”. -
Dissertation Submitted to the Graduate Division of the University of Hawai‘I at Mānoa in Partial Fulfillment of the Requirements for the Degree Of
DEVELOPING THE 60Fe-60Ni SYSTEM FOR EARLY SOLAR SYSTEM CHRONOLOGY A DISSERTATION SUBMITTED TO THE GRADUATE DIVISION OF THE UNIVERSITY OF HAWAI‘I AT MĀNOA IN PARTIAL FULFILLMENT OF THE REQUIREMENTS FOR THE DEGREE OF DOCTOR OF PHILOSOPHY IN GEOLOGY AND GEOPHYSICS AUGUST 2015 By: Myriam Telus Dissertation Committee: Gary R. Huss, Chairperson Kazuhide Nagashima Alexander N. Krot G. Jeffrey Taylor Jonathan P. Williams Keywords: iron, nickel, aluminum, magnesium, radionuclides, meteorites To my husband Nathanael, my endless source of inspiration and joy i ACKNOWLEDGEMENTS I am amazed by how enjoyable graduate school and working toward my Ph.D. has been. For this, I am especially thankful to my advisor Gary Huss. His patience and dedication to helping me grow as a scientist has made this such a rewarding experience. Thanks to all the members of our cosmochemistry group at UH. They are simply the best folks to work with. Thanks to Kazu Nagashima for patiently teaching me to use the ion probe, for working with me on all of my projects and for serving on my dissertation committee. Thanks to Ryan Ogliore for helping me understand proper SIMS data analysis and for traveling all the way to Australia to help with my synchrotron measurements. Thanks to Sasha Krot and Jeff Taylor for serving on my dissertation committee, eagerly discussing ideas with me and for helping me with various aspects of my research. Special thanks to Jonathan Williams, an honorary member of the cosmochemistry group, for hiking all the way from IFA for my dissertation committee meetings. Thanks to Ed Scott for helping with my work on H4 chondrites.