S Low-Altitude Condensed Haze
Total Page:16
File Type:pdf, Size:1020Kb
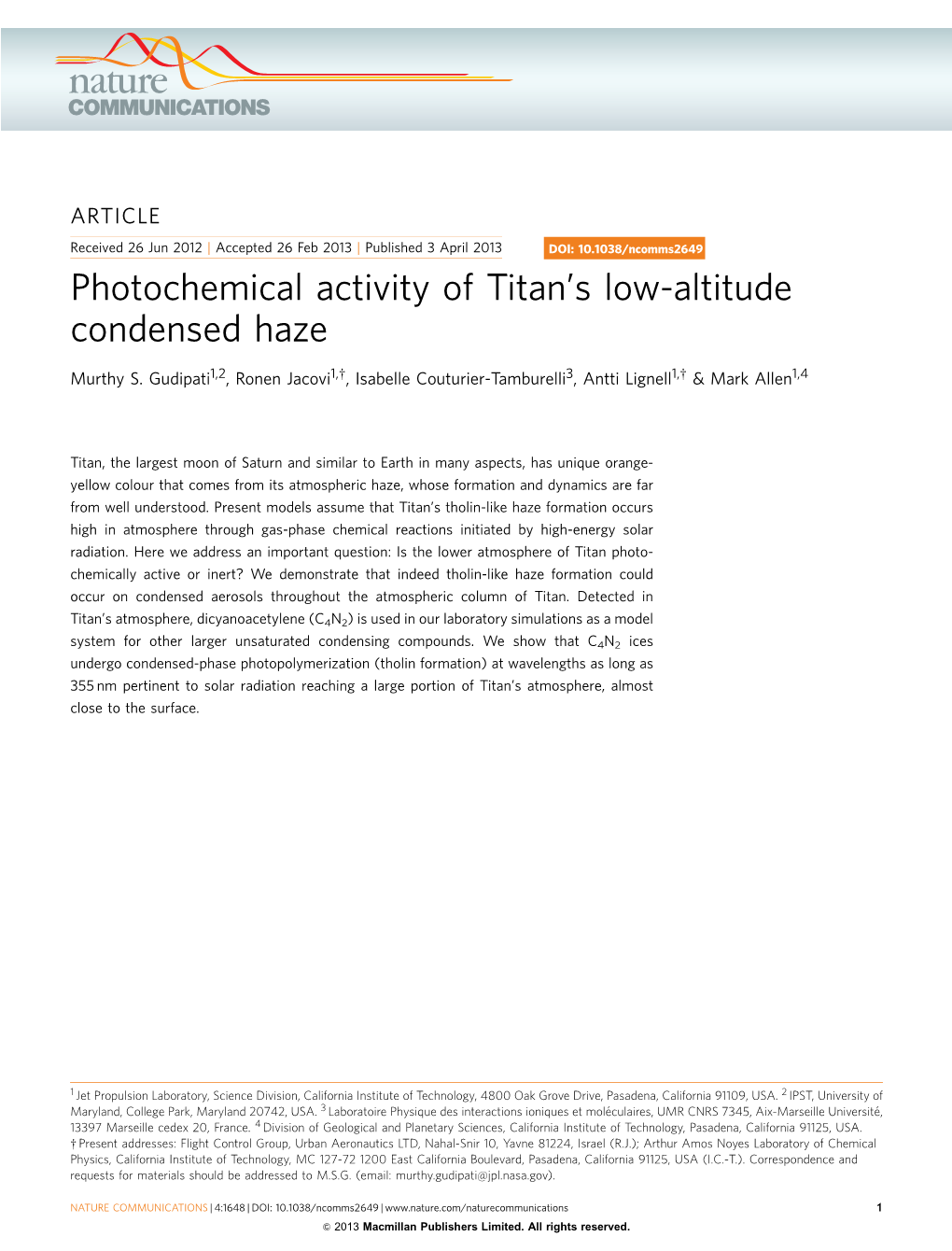
Load more
Recommended publications
-
Interferometric Observations of Large Biologically Interesting Interstellar and Cometary Molecules
SPECIAL FEATURE: PERSPECTIVE Interferometric observations of large biologically interesting interstellar and cometary molecules Lewis E. Snyder* Department of Astronomy, University of Illinois, 1002 West Green Street, Urbana, IL 61801 Edited by William Klemperer, Harvard University, Cambridge, MA, and approved May 26, 2006 (received for review March 3, 2006) Interferometric observations of high-mass regions in interstellar molecular clouds have revealed hot molecular cores that have sub- stantial column densities of large, partly hydrogen-saturated molecules. Many of these molecules are of interest to biology and thus are labeled ‘‘biomolecules.’’ Because the clouds containing these molecules provide the material for star formation, they may provide insight into presolar nebular chemistry, and the biomolecules may provide information about the potential of the associated inter- stellar chemistry for seeding newly formed planets with prebiotic organic chemistry. In this overview, events are outlined that led to the current interferometric array observations. Clues that connect this interstellar hot core chemistry to the solar system can be found in the cometary detection of methyl formate and the interferometric maps of cometary methanol. Major obstacles to under- standing hot core chemistry remain because chemical models are not well developed and interferometric observations have not been very sensitive. Differentiation in the molecular isomers glycolaldehdye, methyl formate, and acetic acid has been observed, but not explained. The extended source structure for certain sugars, aldehydes, and alcohols may require nonthermal formation mechanisms such as shock heating of grains. Major advances in understanding the formation chemistry of hot core species can come from obser- vations with the next generation of sensitive, high-resolution arrays. -
Transition Metal Complexes of Dicyanoacetylene
Louisiana State University LSU Digital Commons LSU Historical Dissertations and Theses Graduate School 1971 Transition Metal Complexes of Dicyanoacetylene: a Study of the Reaction Chemistry of Low Valence States of the Transition Metals, Platinum, Palladium, Nickel, Rhodium, and Iridium. Gregory Lloyd Mcclure Louisiana State University and Agricultural & Mechanical College Follow this and additional works at: https://digitalcommons.lsu.edu/gradschool_disstheses Recommended Citation Mcclure, Gregory Lloyd, "Transition Metal Complexes of Dicyanoacetylene: a Study of the Reaction Chemistry of Low Valence States of the Transition Metals, Platinum, Palladium, Nickel, Rhodium, and Iridium." (1971). LSU Historical Dissertations and Theses. 2001. https://digitalcommons.lsu.edu/gradschool_disstheses/2001 This Dissertation is brought to you for free and open access by the Graduate School at LSU Digital Commons. It has been accepted for inclusion in LSU Historical Dissertations and Theses by an authorized administrator of LSU Digital Commons. For more information, please contact [email protected]. 71-29,382 McCLURE, Gregory Lloyd, 1993- TRANSITION METAL COMPLEXES OF DICYANOACETYLENE: A STUDY OF THE REACTION CHEMISTRY OF LOW VALENCE STATES OF THE TRANSITION METALS, PLATINUM, PALLADIUM, NICKEL, RHODIUM, AND IRIDIUM. The Louisiana State University and Agricultural and Mechanical College, Ph.D., 1971 C hem i stry, inorgan ic University Microfilms, A XEROX Company , Ann Arbor. Michigan THIS DISSERTATION HAS BEEN MICROFILMED EXACTLY AS RECEIVED TRANSITION -
Ionization Photophysics and Spectroscopy of Dicyanoacetylene Sydney Leach, Martin Schwell, Gustavo A
Ionization photophysics and spectroscopy of dicyanoacetylene Sydney Leach, Martin Schwell, Gustavo A. Garcia, Yves Bénilan, Nicolas Fray, Marie-Claire Gazeau, François Gaie-Levrel, Norbert Champion, and Jean-Claude Guillemin Citation: The Journal of Chemical Physics 139, 184304 (2013); doi: 10.1063/1.4826467 View online: http://dx.doi.org/10.1063/1.4826467 View Table of Contents: http://scitation.aip.org/content/aip/journal/jcp/139/18?ver=pdfcov Published by the AIP Publishing This article is copyrighted as indicated in the article. Reuse of AIP content is subject to the terms at: http://scitation.aip.org/termsconditions. Downloaded to IP: 193.51.99.1 On: Wed, 05 Feb 2014 16:48:49 THE JOURNAL OF CHEMICAL PHYSICS 139, 184304 (2013) Ionization photophysics and spectroscopy of dicyanoacetylene Sydney Leach,1,a) Martin Schwell,2,a) Gustavo A. Garcia,3 Yves Bénilan,2 Nicolas Fray,2 Marie-Claire Gazeau,2 François Gaie-Levrel,3,b) Norbert Champion,1 and Jean-Claude Guillemin4 1LERMA UMR CNRS 8112, Observatoire de Paris-Meudon, 5 place Jules-Jansen, 92195 Meudon, France 2LISA UMR CNRS 7583, Université Paris-Est Créteil and Université Paris Diderot, Institut Pierre Simon Laplace, 61 Avenue du Général de Gaulle, 94010 Créteil, France 3Synchrotron SOLEIL, L’Orme des Merisiers, St. Aubin, B.P. 48, 91192 Gif-sur-Yvette Cedex, France 4Institut des Sciences Chimiques de Rennes, Ecole Nationale Supérieure de Chimie de Rennes, CNRS UMR 6226, 11 Allée de Beaulieu, CS 50837, 35708 Rennes Cedex 7, France (Received 17 June 2013; accepted 8 October 2013; published online 11 November 2013) Photoionization of dicyanoacetylene was studied using synchrotron radiation over the excitation range 8–25 eV, with photoelectron-photoion coincidence techniques. -
A Study of the C3H2 Isomers and Isotopologues: first Interstellar Detection of HDCCC?
A&A 586, A110 (2016) Astronomy DOI: 10.1051/0004-6361/201527460 & c ESO 2016 Astrophysics A study of the C3H2 isomers and isotopologues: first interstellar detection of HDCCC? S. Spezzano1;3, H. Gupta2;??, S. Brünken3, C. A. Gottlieb4, P. Caselli1, K. M. Menten5, H. S. P. Müller3, L. Bizzocchi1, P. Schilke3, M. C. McCarthy4, and S. Schlemmer3 1 Max-Planck-Institut für extraterrestrische Physik, Giessenbachstr. 1, 85748 Garching, Germany e-mail: [email protected] 2 California Institute of Technology, 770 S. Wilson Ave., M/C 100-22, Pasadena, CA 91125, USA 3 I. Physikalisches Institut, Universität zu Köln, Zülpicher Str. 77, 50937 Köln, Germany 4 Harvard-Smithsonian Center for Astrophysics, and School of Engineering & Applied Sciences, Harvard University, Cambridge, MA 02138, USA 5 Max-Planck Institut für Radioastronomie, Auf dem Hügel 69, 53121 Bonn, Germany Received 28 September 2015 / Accepted 12 November 2015 ABSTRACT The partially deuterated linear isomer HDCCC of the ubiquitous cyclic carbene (c-C3H2) was observed in the starless cores TMC- 1C and L1544 at 96.9 GHz, and a confirming line was observed in TMC-1 at 19.38 GHz. To aid the identification in these narrow line sources, four centimetre-wave rotational transitions (two in the previously reported Ka = 0 ladder and two new ones in the Ka = 1 ladder) and 23 transitions in the millimetre band between 96 and 272 GHz were measured in high-resolution laboratory spectra. Ten spectroscopic constants in a standard asymmetric top Hamiltonian allow the main transitions of astronomical interest −1 in the Ka ≤ 3 rotational ladders to be calculated to within 0.1 km s in radial velocity up to 400 GHz. -
Abstract Book
Our Astro-Chemical History Past, Present, and Future Sept. 10-14 2018 Assen, The Netherlands Abstract Book Program Monday 12:30-14:00 Lunch 14:00-15:25 14:00 Welcome and logistics 14:10 Summary of activities/results from the Action (Laurent Wiesenfeld) 14:40 The Future of Astrochemistry - Farid Salama (NASA) 15:25-16:00 Coffee break 16:00-17:30 Formation of COMs: surface routes vs new gas-phase routes 16:00 Audrey Coutens (Bordeaux, FR) 16:30 Dimitrios Skouteris (Pisa, IT) 17:00 Alexey Potapov (University ofJena, DE) 17:30-18:30 Welcome reception/posters 19:00-20:00 Dinner Tuesday 9:00-10:30 Low temperature chemistry and kinetics and processes (gas & solid) 9:00 Sergiy Krasnokutskiy (Jena, DE) 9:30 Johannes Kästner (Stuttgart, DE) 10:00 Stanka Jerosimić (Belgrade, RS) 10:30-11:00 Coffee break 11:00-12:30 Isotopic fractionation pathways in space 11:00 Kenji Furuya (Tsukuba, JP) 11:30 Eva Wirström (Chalmers, SE) 12:00 Olli Sipilä (Helsinki, FI; MPE, DE) 12:30-14:00 Lunch 14:00-15:30 Nanoparticles: Condensation, reactivity and diffusion 14:00 Herma Cuppen (Nijmegen, NL) 14:30 David Gobrecht (Leuven, BE) 15:00 Antoni Macià Escatllar (Barcelona, ES) 15:30-16:30 Coffee break/poster session 16:30-18:00 16:30 Chemistry of Planetary Atmospheres - Christiane Helling (St Andrews, UK) 17:15 Comet chemistry - Kathrin Altwegg (Bern, CH) 19:00-20:00 Dinner Wednesday 9:00-11:00 Hydrocarbon chains and rings in space 9:00 Ricardo Urso (INAF, IT) 9:30 Maria Luisa Senent (CSIC, ES) 10:00 Thomas Pino (ISMO Paris, FR) 10:30 Sandra Wiersma (Amsterdam, NL) 11:00-11:30 -
Arxiv:1802.10216V1 [Physics.Chem-Ph] 28 Feb 2018 They Play an Important Role in Their Spectroscopic Characteriza- Not Been Systematically Investigated
Bound and continuum-embedded states of cyanopolyyne anions† Wojciech Skomorowski, Sahil Gulania, and Anna I. Krylova Cyanopolyyne anions were among the first anions discovered in the interstellar medium. The discovery have raised questions about routes of formation of these anions in space. Some of the proposed mechanisms assumed that anionic excited electronic states, either metastable or weakly bound, play a key role in the formation process. Verification of this hypothesis requires detailed knowledge of the electronic states of the anions. Here we investigate bound and continuum states of four cyanopolyyne anions, CN−,C3N−,C5N−, and C7N−, by means of ab initio calculations. We employ the equation-of-motion coupled-cluster method augmented with complex absorbing potential. We predict that already in CN−, the smallest anion in the family, there are several low-lying metastable states of both singlet and triplet spin symmetry. These states, identified as shape resonances, are located between 6.3–8.5 eV above the ground state of the anion (or 2.3–4.5 eV above the ground state of the parent radical) and have widths of a few tenths of eV up to 1 eV. We analyze the identified resonances in terms of leading molecular orbital contributions and Dyson orbitals. As the carbon chain length increases in the C2n+1N− series, these resonances gradually become stabilized and eventually turn into stable valence bound states. The trends in energies of the transitions leading to both resonance and bound excited states can be rationalized by means of the Hückel model. Apart from valence excited states, some of the cyanopolyynes can also support dipole bound states and dipole stabilized resonances, owing to a large dipole moment of the parent radicals in the lowest 2S+ state. -
Laboratory Experiments of Titan Tholins Formed by Photochemistry of Cyanopolyynes
BIO Web of Conferences 2, 01005 (2014) DOI: 10.1051/bioconf/20140201005 C Owned by the authors, published by EDP Sciences, 2014 Laboratory Experiments of Titan Tholins formed by Photochemistry of Cyanopolyynes I. Couturier-Tamburelli1, I. N. Piétri1 1Aix Marseille Université, Laboratoire PIIM, UMR 6633, 13397 Marseille cedex 20 France. Tholins are complex organic materials produced by irradiation of several carbon and nitrogen rich atmosphere. It has been proposed that Tholins could have played an important role in the origin of life on Earth [1]. We investigate the formation of polymer (Tholins) from the photolysis of dicyanoacetylene. As of today, nitriles molecules have been identified in Titan atmosphere. Among these nitriles, the cyanopolyynes (HCnN) are very important since they are the essential constituents in building block amino acids. It is known that a rich phochemistry takes place in the Titan aerosols, and contributes to the evolution of molecular diversity in this atmosphere. These compounds evolve through polymerization processes in aerosol particles, which grow by coagulation and rain down to the surface of Titan containing water ice. We present photochemical processes of larger cyanopolyyne formation from small precursor molecules submitted to long wavelength photons. Under UV irradiation cyanopolyynes are known to induce izomerization process (figure 1) [2] and formation of longer cyanopolyynes [3]. Figure 1: Izomerisation process of cyanopolyynes. We provide the photochemical processes of Titan Tholins formation (figure 2) from cyanopolyyne precursor molecules submitted to long wavelength photons. Such photons penetrate down into the stratosphere and troposphere (figure 3). The photoreactivity of the cyanopolyynes with other Titan molecules are also presented. -
Galactic and Extragalactic Astrochemistry: Heavy-Molecule Precursors to Life?
Astro2020 Science White Paper Galactic and Extragalactic Astrochemistry: Heavy-Molecule Precursors to Life? Thematic Areas: Planetary Systems × Star and Planet Formation Formation and Evolution of Compact Objects Cosmology and Fundamental Physics Stars and Stellar Evolution Resolved Stel lar Populations and their Environments Galaxy Evolution Multi-M essenger Astronomy and Astrophysics Principal Author: Name: Carl Heiles Institution: University of California at Berkeley Email: [email protected] Phone: 510 280 8099 Co-authors: E. D. Araya, WIU; H. Arce, Yale; I. Hoffman, Quest Univ.; Tapasi Ghosh, NRAO; P. Hofner, NMT; S. Kurtz, UNAM; M. Lebron,´ UPR-PR; Hendrik Linz, MPI Heidelberg; L. Olmi, INAF; Y. Pihlstrom,¨ UNM; Chris Salter, NRAO. Abstract: Heavy molecule spectroscopy is a developing subject, with new results from both the terrestrial laboratory and astronomical discovery in the 0.5-10 GHz range where heavy molecule spectral lines are easily distinguished. Dense clouds in space contain an astonishingly rich collection of both familiar and exotic molecules in various states of ionization and excitation. It means that there are many more ways to build large organic molecules in these environments than have been previously explored. These add to the number of paths available for making the complex organic molecules and other large molecular species that may be the precursors to life. 1 1 Heavy Molecules Most known interstellar molecules are small and have their lowest rotational transitions in the millimeter wavelength range (e.g., McGuire 2018a). Heavier molecules have their lowest rota- tional transitions in the microwave range and are especially prominent at the low temperatures that characterize many dense molecular clouds. -
The Interstellar Chemistry of C3H and C3H2 Isomers
The interstellar chemistry of C3H and C3H2 isomers. Jean-Christophe Loison1*, Marcelino Agúndez2, Valentine Wakelam3, Evelyne Roueff4, Pierre Gratier3, Núria Marcelino5, Dianailys Nuñez Reyes1, José Cernicharo2, Maryvonne Gerin6. *Corresponding author: [email protected] 1 Institut des Sciences Moléculaires (ISM), CNRS, Univ. Bordeaux, 351 cours de la Libération, 33400, Talence, France 2 Instituto de Ciencia de Materiales de Madrid, CSIC, C\ Sor Juana Inés de la Cruz 3, 28049 Cantoblanco, Spain 3 Laboratoire d'astrophysique de Bordeaux, Univ. Bordeaux, CNRS, B18N, allée Geoffroy Saint-Hilaire, 33615 Pessac, France. 4 LERMA, Observatoire de Paris, PSL Research University, CNRS, Sorbonne Universités, UPMC Univ. Paris 06, F-92190 Meudon, France 5 INAF, Osservatorio di Radioastronomia, via P. Gobetti 101, 40129 Bologna, Italy 6 LERMA, Observatoire de Paris, PSL Research University, CNRS, Sorbonne Universités, UPMC Univ. Paris 06, Ecole Normale Supérieure, F-75005 Paris, France We report the detection of linear and cyclic isomers of C3H and C3H2 towards various starless cores and review the corresponding chemical pathways involving neutral (C3Hx with x=1,2) + and ionic (C3Hx with x = 1,2,3) isomers. We highlight the role of the branching ratio of + + electronic Dissociative Recombination (DR) reactions of C3H2 and C3H3 isomers showing * * that the statistical treatment of the relaxation of C3H and C3H2 produced in these DR reactions may explain the relative c,l-C3H and c,l-C3H2 abundances. We have also introduced in the model the third isomer of C3H2 (HCCCH). The observed cyclic-to-linear C3H2 ratio vary from 110 ± 30 for molecular clouds with a total density around 1×104 molecules.cm-3 to 30 ± 10 for molecular clouds with a total density around 4×105 molecules.cm-3, a trend well reproduced with our updated model. -
Chemical Modeling of Interstellar Molecules in Dense Cores
Chemical Modeling of Interstellar Molecules in Dense Cores Dissertation Presented in Partial Fulfillment of the Requirements for the Degree Doctor of Philosophy in the Graduate School of The Ohio State University By Donghui Quan, M.S. Graduate Program in Chemical Physics The Ohio State University 2009 Dissertation Committee: Professor Eric Herbst, Advisor Professor Frank C. De Lucia Professor Anil K. Pradhan Copyright by Donghui Quan 2009 ii Abstract There are billions of stars in our galaxy, the Milky Way Galaxy. In between the stars is where the so-called “interstellar medium” locates. The majority of the mass of interstellar medium is clumped into interstellar clouds, in which cold and hot dense cores exist. Despite of the extremely low densities and low temperatures of the dense cores, over one hundred molecules have been found in these sources. This makes the field of astrochemistry vivid. Chemical modeling plays very important roles to understand the mechanism of formation and destruction of interstellar molecules. In this thesis, chemical kinetics models of different types were applied: in Chapter 4, pure gas phase models were used for seven newly detected or confirmed molecules by the Green Bank Telescope; in Chapter 5, the potential reason of non-detection of O2 was explored; in Chapter 6, the mysterious behavior of CHNO and CHNS isomers were studied by gas-grain models. In addition, effects of varying rate coefficients to the models are also discussed in Chapter 3 and 7. ii Dedication Dedicated to my parents Quan He (全和), Li Lianxiang (李廉祥) and my wife Wang Jing (王璟) iii Acknowledgements First of all, I would like to thank my advisor, Professor Eric Herbst, who has always been supportive in all means: a mentor in my graduate study, a guide in astrochemical research, and a kind “father” in the life. -
Polymerization of Dicyanoacetylene † ‡ ○ † ○ ■ ¶ § ■ § ■ Huiyang Gou,*, , , Li Zhu, , Haw-Tyng Huang, , Arani Biswas, , Derek W
Article pubs.acs.org/cm From Linear Molecular Chains to Extended Polycyclic Networks: Polymerization of Dicyanoacetylene † ‡ ○ † ○ ■ ¶ § ■ § ■ Huiyang Gou,*, , , Li Zhu, , Haw-Tyng Huang, , Arani Biswas, , Derek W. Keefer, , ∥ ◆ ⊥ † ‡ † ‡ † Brian L. Chaloux, , Clemens Prescher, Liuxiang Yang, , Duck Young Kim, , Matthew D. Ward, ■ # # ∇ ∥ § ■ ¶ □ Jordan Lerach, Shengnan Wang, Artem R. Oganov, , Albert Epshteyn, John V. Badding, , , , † and Timothy A. Strobel*, † Geophysical Laboratory, Carnegie Institution of Washington, Washington, DC 20015, United States ‡ Center for High Pressure Science and Technology Advanced Research, Beijing 100094, China § Department of Chemistry, The Pennsylvania State University, University Park, Pennsylvania 16802, United States ■ Materials Research Institute, The Pennsylvania State University, University Park, Pennsylvania 16802, United States ¶ Department of Materials Science and Engineering, The Pennsylvania State University, University Park, Pennsylvania 16802, United States □ Department of Physics, The Pennsylvania State University, University Park, Pennsylvania 16802, United States ∥ Naval Research Laboratory, 4555 Overlook Avenue, SW, Washington, DC 20375, United States ⊥ Center for Advanced Radiation Sources, University of Chicago, Argonne, Illinois 60437, United States # Department of Geosciences, Center for Materials by Design, and Institute for Advanced Computational Science, State University of New York, Stony Brook, New York 11794-2100, United States ∇ Skolkovo Institute of Science and Technology, -
The Center for the Study of Terrestrial and Extraterrestrial Atmospheres (CSTEA) Dr
_ ; i •_ '. : .' _ ";¢ hi-__¸_:_7 NASA-CR-204199 The Center For The Study Of Terrestrial And Extraterrestrial Atmospheres (CSTEA) Dr. Arthur N. Thorpe, Director Dr. Vernon R. Morris, Deputy Director Funded By The National Aeronautics And Space Administration (NASA) NAGW 2950 Five-Year Report April 1992-December 1996 Howard University 2216 6th Street, NW Room 103 Washington, DC 20059 202-806-5172 202-806-4430 (FAX) URL Home Page: http://www.cstea.howard.edu e-mail: thorpe@ cstea.cstea.howard.edu TABLE OF CONTENTS CSTEA's Existence ... Then And Now 1 The CSTEA PIs ... Their Research And Students 6 Dr. Peter Bainum ... 7 Dr. Anand Batra ... 10 Dr. Robert Catchings ... 10 Dr. L. Y. Chiu ... 11 Dr. Balaram Dey ... 13 Dr. Joshua Halpern ... 14 Dr. Peter Hambright ... 20 Dr. Gary Harris ... 24 Dr. Lewis Klein ... 26 Dr. Cidambi Kumar ... 28 Dr. James Lindesay ... 29 Dr. Prabhakar Misra ... 32 Dr. Vernon Morris ... 35 Dr. Hideo Okabe ... 39 Dr. Steven Pollack ... 41 Dr. Steven Richardson ... 42 Dr. Yehuda Salu ... 43 Dr. Sonya Smith ... 45 Dr. Michael Spencer ... 46 Dr. George Morgenthaler ... 48 Five Year Report (April 1992-31 December 1996) Center for the Study of Terrestrial and Extraterrestrial Atmospheres (CSTEA) Dr. Arthur N. Thorpe, Director CSTEA's EXISTENCE ... THEN AND NOW The Center for the Study of Terrestrial and Extraterrestrial Atmospheres (CSTEA) was established in 1992 by a grant from the National Aeronautics and Space Administra- tion (NASA) Minority University Research and Education Division (MURED). Since CSTEA was first proposed in October of 1991 by Dr. William Gates, then Chairman of the Department of Physics at Howard University, it has become a world-class, comprehen- sive, nationally competitive university center for atmospheric research ..