Antibiotic Sensitivity Screening of Klebsiella Spp. and Raoultella Spp
Total Page:16
File Type:pdf, Size:1020Kb
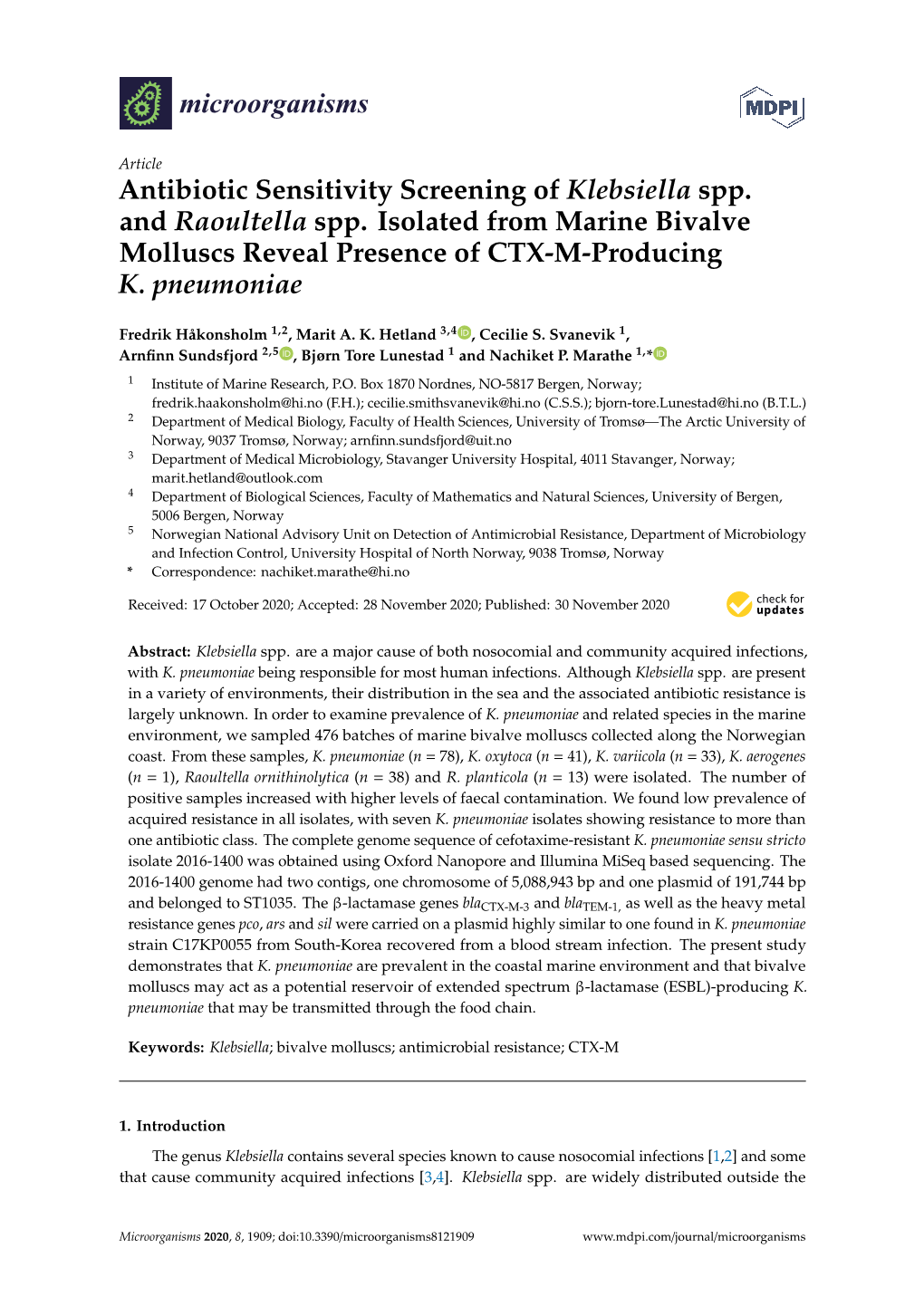
Load more
Recommended publications
-
Pseudomonas Spp. and Other Psychrotrophic
Pesq. Vet. Bras. 39(10):807-815, October 2019 DOI: 10.1590/1678-5150-PVB-6037 Original Article Livestock Diseases ISSN 0100-736X (Print) ISSN 1678-5150 (Online) PVB-6037 LD Pseudomonas spp. and other psychrotrophic microorganisms in inspected and non-inspected Brazilian Minas Frescal cheese: proteolytic, lipolytic and AprX production potential1 Pedro I. Teider Junior2, José C. Ribeiro Júnior3* , Eric H. Ossugui2, Ronaldo Tamanini2, Juliane Ribeiro2, Gislaine A. Santos2 2 and Vanerli Beloti2 , Amauri A. Alfieri ABSTRACT.- Teider Junior P.I., Ribeiro Júnior J.C., Ossugui E.H., Tamanini R., Ribeiro J., Santos G.A., Pseudomonas spp. and other psychrotrophic microorganisms Pseudomonas spp. and other psychrotrophic in inspected and non-inspected Brazilian Minas Frescal cheese: proteolytic, lipolytic andAlfieri AprX A.A. production& Beloti V. 2019. potential . Pesquisa Veterinária Brasileira 39(10):807-815. Instituto microorganisms in inspected and non- Nacional de Ciência e Tecnologia para a Cadeia Produtiva do Leite, Universidade Estadual de inspected Brazilian Minas Frescal cheese: Londrina, Rodovia Celso Garcia Cid PR-445 Km 380, Cx. Postal 10.011, Campus Universitário, proteolytic, lipolytic and AprX production Londrina, PR 86057-970, Brazil. E-mail: [email protected] The most consumed cheese in Brazil, Minas Frescal cheese (MFC) is highly susceptible to potential microbial contamination and clandestine production and commercialization can pose a risk to consumer health. The storage of this fresh product under refrigeration, although more appropriate, may favor the growth of spoilage psychrotrophic bacteria. The objective of this [Pseudomonas spp. e outros micro-organismos study was to quantify and compare Pseudomonas spp. and other psychrotrophic bacteria in inspected and non-inspected MFC samples, evaluate their lipolytic and proteolytic activities and e não inspecionados: potencial proteolítico, lipolítico e their metalloprotease production potentials. -
516278V1.Full.Pdf
bioRxiv preprint doi: https://doi.org/10.1101/516278; this version posted January 9, 2019. The copyright holder for this preprint (which was not certified by peer review) is the author/funder. All rights reserved. No reuse allowed without permission. 1 1 Description of Klebsiella africanensis sp. nov., 2 Klebsiella variicola subsp. tropicalensis subsp. nov. 3 and Klebsiella variicola subsp. variicola subsp. nov. 4 5 Carla Rodriguesa†, Virginie Passeta†, Andriniaina Rakotondrasoab, Thierno Abdoulaye 6 Dialloc, Alexis Criscuolod & Sylvain Brissea,* 7 8 a Institut Pasteur, Biodiversity and Epidemiology of Bacterial Pathogens, Paris, France 9 b Institut Pasteur Madagascar, Antananarivo, Madagascar 10 c Institut Pasteur of Dakar, Senegal 11 d Institut Pasteur, Bioinformatics and Biostatistics Hub, C3BI, USR 3756 IP CNRS, Paris, 12 France. 13 14 † These authors contributed equally to this work. 15 16 E-mail addresses: [email protected] (C. Rodrigues), 17 [email protected] (V. Passet), [email protected] (A. Rakotondrasoa), 18 [email protected] (T. A. Diallo), [email protected] (A. Criscuolo), 19 [email protected] (S. Brisse) “*Correspondence and reprints” 20 bioRxiv preprint doi: https://doi.org/10.1101/516278; this version posted January 9, 2019. The copyright holder for this preprint (which was not certified by peer review) is the author/funder. All rights reserved. No reuse allowed without permission. 2 21 Abstract 22 The bacterial pathogen Klebsiella pneumoniae comprises several phylogenetic groups 23 (Kp1 to Kp7), two of which (Kp5 and Kp7) have no taxonomic status. Here we show that 24 group Kp5 is closely related to Klebsiella variicola (Kp3), with an average nucleotide identity 25 (ANI) of 96.4%, and that group Kp7 has an ANI of 94.7% with Kp1 (K. -
Succession and Persistence of Microbial Communities and Antimicrobial Resistance Genes Associated with International Space Stati
Singh et al. Microbiome (2018) 6:204 https://doi.org/10.1186/s40168-018-0585-2 RESEARCH Open Access Succession and persistence of microbial communities and antimicrobial resistance genes associated with International Space Station environmental surfaces Nitin Kumar Singh1, Jason M. Wood1, Fathi Karouia2,3 and Kasthuri Venkateswaran1* Abstract Background: The International Space Station (ISS) is an ideal test bed for studying the effects of microbial persistence and succession on a closed system during long space flight. Culture-based analyses, targeted gene-based amplicon sequencing (bacteriome, mycobiome, and resistome), and shotgun metagenomics approaches have previously been performed on ISS environmental sample sets using whole genome amplification (WGA). However, this is the first study reporting on the metagenomes sampled from ISS environmental surfaces without the use of WGA. Metagenome sequences generated from eight defined ISS environmental locations in three consecutive flights were analyzed to assess the succession and persistence of microbial communities, their antimicrobial resistance (AMR) profiles, and virulence properties. Metagenomic sequences were produced from the samples treated with propidium monoazide (PMA) to measure intact microorganisms. Results: The intact microbial communities detected in Flight 1 and Flight 2 samples were significantly more similar to each other than to Flight 3 samples. Among 318 microbial species detected, 46 species constituting 18 genera were common in all flight samples. Risk group or biosafety level 2 microorganisms that persisted among all three flights were Acinetobacter baumannii, Haemophilus influenzae, Klebsiella pneumoniae, Salmonella enterica, Shigella sonnei, Staphylococcus aureus, Yersinia frederiksenii,andAspergillus lentulus.EventhoughRhodotorula and Pantoea dominated the ISS microbiome, Pantoea exhibited succession and persistence. K. pneumoniae persisted in one location (US Node 1) of all three flights and might have spread to six out of the eight locations sampled on Flight 3. -
Neonatal Septicemia Caused by a Rare Pathogen: Raoultella Planticola
Chen et al. BMC Infectious Diseases (2020) 20:676 https://doi.org/10.1186/s12879-020-05409-5 CASE REPORT Open Access Neonatal septicemia caused by a rare pathogen: Raoultella planticola - a report of four cases Xianrui Chen1,2,3, Shaoqing Guo1,2,3* , Dengli Liu1,2,3 and Meizhen Zhong1,2,3 Abstract Background: Raoultella planticola(R.planticola) is a very rare opportunistic pathogen and sometimes even associated with fatal infection in pediatric cases. Recently,the emergence of carbapenem resistance strains are constantly being reported and a growing source of concern for pediatricians. Case presentation: We reported 4 cases of neonatal septicemia caused by Raoultella planticola. Their gestational age was 211 to 269 days, and their birth weight was 1490 to 3000 g.The R. planticola infections were detected on the 9th to 27th day after hospitalizationandoccuredbetweenMayandJune.They clinically manifested as poor mental response, recurrent cyanosis, apnea, decreased heart rate and blood oxygen, recurrent jaundice, fever or nonelevation of body temperature. The C-reactive protein and procalcitonin were elevated at significantly in the initial phase of the infection,and they had leukocytosis or leukopenia. Prior to R.planticola infection,all of them recevied at least one broad-spectrum antibiotic for 7- 27d.All the R.planticola strains detected were only sensitive to amikacin, but resistant to other groups of drugs: cephalosporins (such as cefazolin, ceftetan,etc) and penicillins (such as ampicillin-sulbactam,piperacillin, etc),and even developed resistance to carbapenem. All the infants were clinically cured and discharged with overall good prognosis. Conclusion: Neonatal septicemia caused by Raoultella planticola mostly occured in hot and humid summer, which lack specific clinical manifestations. -
Identification of Raoultella Terrigena As a Rare Causative Agent of Subungual Abscess Based on 16S Rrna and Housekeeping Gene Sequencing
Hindawi Publishing Corporation Canadian Journal of Infectious Diseases and Medical Microbiology Volume 2016, Article ID 3879635, 4 pages http://dx.doi.org/10.1155/2016/3879635 Case Report Identification of Raoultella terrigena as a Rare Causative Agent of Subungual Abscess Based on 16S rRNA and Housekeeping Gene Sequencing Yu Wang,1 Xiawei Jiang,1 Zemin Xu,2 Chaoqun Ying,1 Wei Yu,1 and Yonghong Xiao1 1 State Key Laboratory for Diagnosis and Treatment of Infectious Diseases, Collaborative Innovation Center for Diagnosis and Treatment of Infectious Diseases, The First Affiliated Hospital, School of Medicine, Zhejiang University, Hangzhou, China 2Ningbo Institute of Microcirculation and Henbane, Ningbo, China Correspondence should be addressed to Yonghong Xiao; [email protected] Received 8 October 2015; Revised 28 April 2016; Accepted 15 May 2016 Academic Editor: Maurizio Sanguinetti Copyright © 2016 Yu Wang et al. This is an open access article distributed under the Creative Commons Attribution License, which permits unrestricted use, distribution, and reproduction in any medium, provided the original work is properly cited. A 63-year-old-man was admitted to our hospital with severe subungual abscess. Bacteria were isolated from pus samples, and an inconsistent identification was shown by VITEK 2 system and MALDI-TOF mass spectrometry as Raoultella planticola and Raoultella terrigena, respectively. Molecular identification by 16S rRNA sequencing suggested that the isolate is R. terrigena,and this was further demonstrated by sequencing three housekeeping genes (rpoB, gyrA,andparC) with phylogenetic analysis. To our knowledge, this is the first report of subungual abscess caused by R. terrigena, a rare case of human infection due to soil bacterium. -
CTX-M-9 Group ESBL-Producing Raoultella Planticola Nosocomial
Tufa et al. Ann Clin Microbiol Antimicrob (2020) 19:36 https://doi.org/10.1186/s12941-020-00380-0 Annals of Clinical Microbiology and Antimicrobials CASE REPORT Open Access CTX-M-9 group ESBL-producing Raoultella planticola nosocomial infection: frst report from sub-Saharan Africa Tafese Beyene Tufa1,2,3*† , Andre Fuchs2,3†, Torsten Feldt2,3, Desalegn Tadesse Galata1, Colin R. Mackenzie4, Klaus Pfefer4 and Dieter Häussinger2,3 Abstract Background: Raoultella are Gram-negative rod-shaped aerobic bacteria which grow in water and soil. They mostly cause nosocomial infections associated with surgical procedures. This case study is the frst report of a Raoultella infec- tion in Africa. Case presentation We report a case of a surgical site infection (SSI) caused by Raoultella planticola which developed after caesarean sec- tion (CS) and surgery for secondary small bowel obstruction. The patient became febrile with neutrophilia (19,157/µL) 4 days after laparotomy and started to develop clinical signs of a SSI on the 8 th day after laparotomy. The patient con- tinued to be febrile and became critically ill despite empirical treatment with ceftriaxone and vancomycin. Raoultella species with extended antimicrobial resistance (AMR) carrying the CTX-M-9 β-lactamase was isolated from the wound discharge. Considering the antimicrobial susceptibility test, ceftriaxone was replaced by ceftazidime. The patient recovered and could be discharged on day 29 after CS. Conclusions: Raoultella planticola was isolated from an infected surgical site after repeated abdominal surgery. Due to the infection the patient’s stay in the hospital was prolonged for a total of 4 weeks. It is noted that patients under- going surgical and prolonged inpatient treatment are at risk for infections caused by Raoultella. -
Pathogenic Significance of Klebsiella Oxytoca in Acute Respiratory Tract Infection
Thorax 1983;38:205-208 Thorax: first published as 10.1136/thx.38.3.205 on 1 March 1983. Downloaded from Pathogenic significance of Klebsiella oxytoca in acute respiratory tract infection JOAN T POWER, MARGARET-A CALDER From the Department ofRespiratory Medicine and the Bacteriology Laboratory, City Hospital, Edinburgh ABSTRACT A retrospective study of all Klebsiella isolations from patients admitted to hospital with acute respiratory tract infections over a 27-month period was carried out. Ten of the Klebsiella isolations from sputum and one from a blood culture were identified as Klebsiella oxytoca. The clinical and radiological features of six patients are described. Four of these patients had lobar pneumonia, one bronchopneumonia, and one acute respiratory tract infection superimposed on cryptogenic fibrosing alveolitis. One of the patients with lobar pneumonia had a small-cell carcinoma of the bronchus. We concluded that Klebsiella oxytoca was of definite pathogenic significance in these six patients and of uncertain significance in the remaining five patients. Klebsiella oxytoca has not previously been described as a specific pathogen in the respiratory tract. Close co-operation between clinicians and microbiologists in the management of patients with respiratory infections associated with the Enterobacteriaceae is desirable. Klebsiella oxytoca has not previously been described agar plate was inoculated and incubated for 18 copyright. as a specific respiratory pathogen. Bacillus oxytoca hours. The API 20E system (Analytal Product Inc) was first isolated by Flugge from a specimen of sour was used to identify the biochemical reactions. The milk in 1886.' 2 It was not until 1963 that the organ- two biochemical reactions which differentiate Kleb- ism was accepted as a member of the genus Kleb- siella oxytoca from the Klebsiella pneumoniae organ- siella and then only with reluctance on the part of ism are its ability to liquify gelatin and its indole http://thorax.bmj.com/ some authorities.3 To define more clearly the role of positivity. -
Klebsiella Pneumoniae Ar-Bank#0453
KLEBSIELLA PNEUMONIAE AR-BANK#0453 Key RESISTANCE: KPC MIC (µg/ml) RESULTS AND INTERPRETATION PROPAGATION DRUG MIC INT DRUG MIC INT MEDIUM Amikacin 32 I Colistin 0.5 --- Ampicillin >32 R Doripenem >8 R Medium: Trypticase Soy Agar with 5% Sheep Blood (BAP) Ampicillin/sulbactam1 >32 R Ertapenem >8 R GROWTH CONDITIONS Aztreonam >64 R Gentamicin 1 S Temperature: 35⁰C Cefazolin >8 R Imipenem >64 R Atmosphere: Aerobic 2 Cefepime >32 R Imipenem+chelators >32 --- PROPAGATION Levofloxacin PROCEDURE Cefotaxime >64 R >8 R Cefotaxime/clavulanic 1 >32 --- Meropenem >8 R Remove the sample vial to a acid container with dry ice or a Cefoxitin >16 R Piperacillin/tazobactam1 >128 R freezer block. Keep vial on ice Ceftazidime >128 R Polymyxin B 0.5 --- or block. (Do not let vial content thaw) Ceftazidime/avibactam1 16 R3 Tetracycline 8 I Ceftazidime/clavulanic >64 --- Tigecycline 1 S3 Open vial aseptically to avoid acid1 contamination Ceftriaxone >32 R Tobramycin 16 R 1 Using a sterile loop, remove a Ciprofloxacin >8 R Trimethoprim/sulfamethoxazole >8 R small amount of frozen isolate from the top of the vial S – I –R Interpretation (INT) derived from CLSI 2016 M100 S26 1 Reflects MIC of first component Aseptically transfer the loop 2 Screen for metallo-beta-lactamase production to BAP [Rasheed et al. Emerging Infectious Diseases. 2013. 19(6):870-878] 3 Based on FDA break points Use streak plate method to isolate single colonies Incubate inverted plate at 35⁰C for 18-24 hrs. [email protected] http://www.cdc.gov/drugresistance/resistance-bank/ BIOSAFETY LEVEL 2 Appropriate safety procedures should always be used with this material. -
Klebsiella Pneumoniae: Virulence, Biofilm and Antimicrobial Resistance
Divya Bharathi PIDJ ESPID REPORTS AND REVIEWS PIDJ-217-384 CONTENTS Klebsiella pneumoniae Vitulence, Biofilm and Resistance in Klebsiella EDITORIAL BOARD Piperaki et al Editor: Delane Shingadia Board Members David Burgner (Melbourne, Cristiana Nascimento-Carvalho George Syrogiannopoulos XXX Australia) (Bahia, Brazil) (Larissa, Greece) Kow-Tong Chen (Tainan,Taiwan) Ville Peltola (Turku, Finland) Tobias Tenenbaum (Mannhein, Germany) Luisa Galli (Florence, Italy) Emmanuel Roilides (Thessaloniki, Marc Tebruegge (Southampton, UK) Steve Graham (Melbourne, Pediatr Infect Dis J Greece) Marceline Tutu van Furth (Amsterdam, Australia) Ira Shah (Mumbai, India) The Netherlands) Lippincott Williams & Wilkins Klebsiella pneumoniae: Virulence, Biofilm and Antimicrobial Hagerstown, MD Resistance Evangelia-Theophano Piperaki, MD, PhD,* George A. Syrogiannopoulos, MD, PhD,† Leonidas S. Tzouvelekis, MD, PhD,* and George L. Daikos, MD, PhD‡ Key Words: Klebsiella pneumoniae, virulence, ingitis in premature neonates and infants as the immune response through cytokine and biofilm, resistance well as serious infections in immunocompro- chemokine production (Fig. 1). Among the mised and malnourished children, whereas effector cells that are recruited first to the in the community, K. pneumoniae is a com- infection site are the neutrophils. Impor- mon cause of urinary tract infections among tant mediators involved in this process are lebsiella pneumoniae is a ubiquitous immunocompetent children. interleukin (IL)-8 and IL-23, which induces K Gram-negative encapsulated bacterium In recent years, most K. pneumoniae production of IL-17 that promotes granu- that resides in the mucosal surfaces of mam- infections are caused by strains termed “clas- lopoietic response.6-7 IL-12 also amplifies the mals and the environment (soil, water, etc.). sic” K. pneumoniae (cKp). -
Severe Pansinusitis Due to Raoultella Ornithinolytica
American Journal of Infectious Diseases Case Report Severe Pansinusitis Due to Raoultella Ornithinolytica Mitchell R. Gore Department of Otolaryngology, State University of New York-Upstate Medical University, Syracuse, NY, USA Article history Abstract: Raoultella ornithinolytica is a gram -negative aerobic bacterium Received: 23-09-2017 typically found in plants, soil and water. Reports of Raoultella Revised: 05-12-2017 ornithinolytica causing infections in humans, especially in the head and Accepted: 20-12-2017 neck, are rare. Herein we report a case of a woman with severe septal deviation, chronic headaches and facial pain who was revealed to have Tel: +1 315 492 3741 Fax: +1 315 492 5791 Raoultella ornithinolytica pansinusitis incidentally noted during an Email: [email protected] endoscopic septoplasty. Raoultella ornithinolytica was isolated from cultures of the diffuse purulent drainage found in her maxillary and ethmoid sinuses. This case indicates that while a rare cause of head and neck infection in humans, Raoultella ornithinolytica can be found as the causative pathogen in severe sinusitis. Keywords: Raoultella ornithinolytica , Sinusitis, Infection Introduction distress. Head and neck and cranial nerve examinations were normal. Nasal endoscopy revealed severe bilateral Raoultella ornithinolytica is a member of a genus of inferior turbinate hypertrophy and severe septal deviation Gram-negative, oxidase-negative, aerobic, nonmotile, causing bilateral nasal obstruction. The inferior turbinate capsulated, facultatively anaerobic rods closely related to hypertrophy and septal deviation were so significant that the Klebsiella genus in the Enterobacteriaceae family. the middle meatus could not be visualized. Given the The genus is named after noted French bacteriologist patient’s complaints she was counseled on the risks, Didier Raoult (Drancourt et al. -
Complete Genome Sequence of Endophytic Nitrogen-Fixing
Lin et al. Standards in Genomic Sciences (2015) 10:22 DOI 10.1186/s40793-015-0004-2 SHORT GENOME REPORT Open Access Complete genome sequence of endophytic nitrogen-fixing Klebsiella variicola strain DX120E Li Lin1†, Chunyan Wei2†, Mingyue Chen3†, Hongcheng Wang3, Yuanyuan Li3, Yangrui Li1,2, Litao Yang1,2,4* and Qianli An3* Abstract Klebsiella variicola strain DX120E (=CGMCC 1.14935) is an endophytic nitrogen-fixing bacterium isolated from sugarcane crops grown in Guangxi, China and promotes sugarcane growth. Here we summarize the features of the strain DX120E and describe its complete genome sequence. The genome contains one circular chromosome and two plasmids, and contains 5,718,434 nucleotides with 57.1% GC content, 5,172 protein-coding genes, 25 rRNA genes, 87 tRNA genes, 7 ncRNA genes, 25 pseudo genes, and 2 CRISPR repeats. Keywords: Endophyte, Klebsiella variicola, Klebsiella pneumoniae, Nitrogen fixation, Pathogenicity, Plant growth-promoting bacteria, Sugarcane Introduction roots and shoots, to fix N2 in association with sugarcane The species Klebsiella variicola was classified in 2004 plants, and to promote sugarcane growth [10], and and consisted of clinical and plant-associated isolates thusshowsapotentialasabiofertilizer.Herewe [1].The species K. singaporensis was classified in 2004 present a summary of the features of the K. variicola based on a single soil isolate [2] and was recently identi- strain DX120E (=CGMCC 1.14935) and its complete fied as a later junior heterotypic synonym of K. variicola genome sequence, and thus provide a genetic back- [3]. K. variicola is able to fix N2 [1]. K. variicola strain ground to understand its endophytic lifestyle, plant At-22, one of the dominant bacteria in the fungus gar- growth-promoting potentials, and similarities and dens of leaf-cutter ants, provides nitrogen source by N2 differences to other plant-associated and clinical K. -
Comparison Between Vitek MS, Bruker Biotyper, Vitek2, and API20E for Differentiation of Species of the Genus Raoultella
European Journal of Clinical Microbiology & Infectious Diseases (2019) 38:467–470 https://doi.org/10.1007/s10096-018-03444-4 ORIGINAL ARTICLE Comparison between Vitek MS, Bruker Biotyper, Vitek2, and API20E for differentiation of species of the genus Raoultella Carlos Ruiz de Alegría Puig1 & Marina Fernández Torres1 & Eduardo Marfil-Pérez2,3 & María Isabel Rodríguez Ferández1 & Manuel Causse Del Río2,3 & Jesús Agüero Balbín1,4 & Luis Martínez-Martínez2,3,5 Received: 11 September 2018 /Accepted: 29 November 2018 /Published online: 25 January 2019 # Springer-Verlag GmbH Germany, part of Springer Nature 2019 Abstract Rapid and reliable identification of microorganisms in the clinical laboratory is essential for an early and accurate diagnosis guiding timely therapy. However, conventional methods are sometimes unreliable and show controversial outcomes. Matrix- assisted laser desorption/ionization time-of-flight mass spectrometry (MALDI-TOF MS) has been reported as a rapid and reliable method for identification of bacteria and fungi isolated from clinical samples. Members of the genus Raoultella are increasingly recognized as clinically relevant. There are difficulties in their identification at the species level since sequencing the 16S rRNA or the rpoB genes does not show conclusive results. The aim of this study has been to compare two MALDI-TOF MS systems (Vitek MS and Bruker Biotyper) with Vitek2 and API20E systems for differentiation of Raoultella species. A collection of 97 clinical isolates of Raoultella species was identified with Vitek MS, in parallel with Vitek2 and API, and finally with Bruker Biotyper. Among the two most widely used MALDI-TOF MS platforms, results obtained with Vitek MS were slightly superior to those obtained with the Bruker Biotyper system, with sensitivities and specificities of 98.9/57.9% and 98.8/37.0%, respectively.