Proteomics Profiling and Pathway Analysis of Hippocampal Aging In
Total Page:16
File Type:pdf, Size:1020Kb
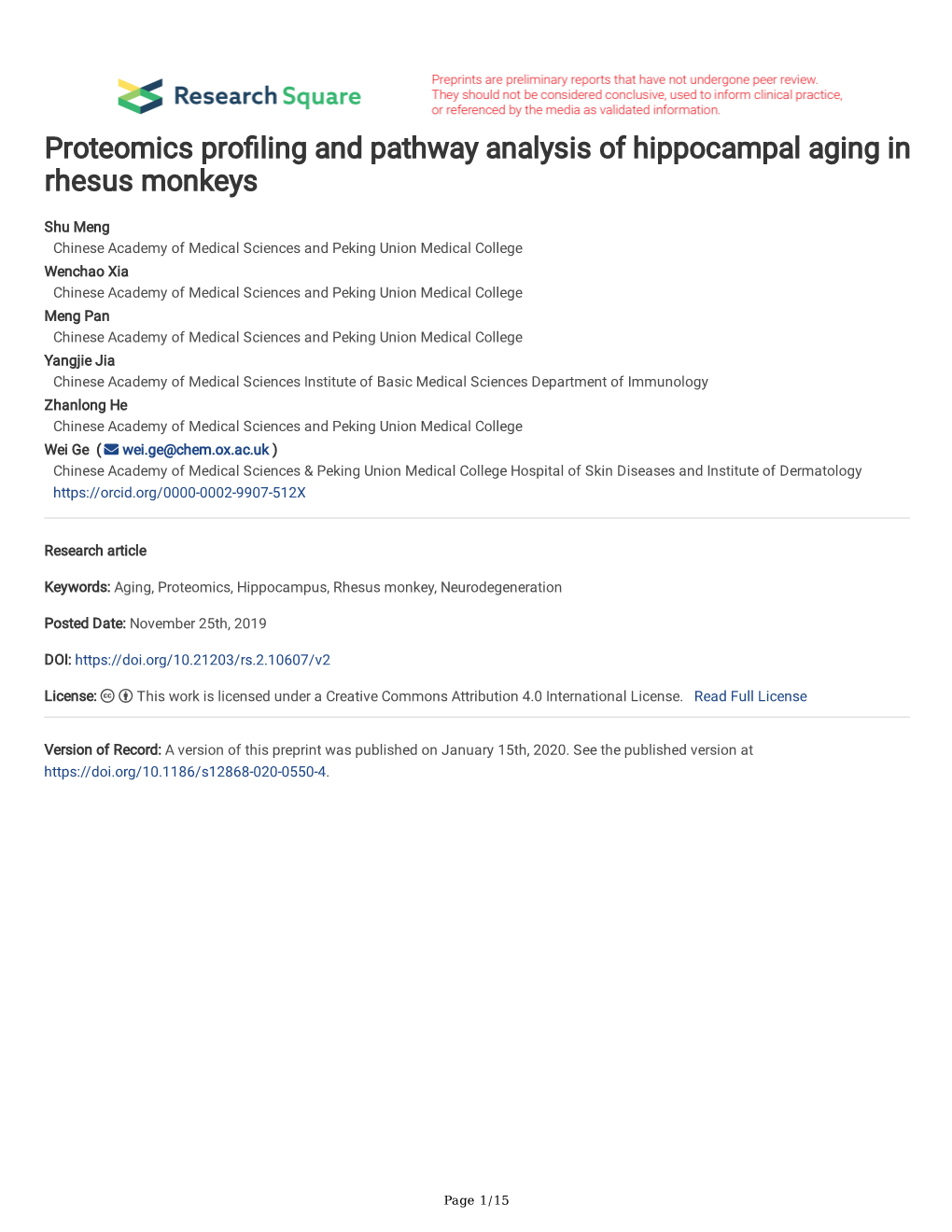
Load more
Recommended publications
-
A Computational Approach for Defining a Signature of Β-Cell Golgi Stress in Diabetes Mellitus
Page 1 of 781 Diabetes A Computational Approach for Defining a Signature of β-Cell Golgi Stress in Diabetes Mellitus Robert N. Bone1,6,7, Olufunmilola Oyebamiji2, Sayali Talware2, Sharmila Selvaraj2, Preethi Krishnan3,6, Farooq Syed1,6,7, Huanmei Wu2, Carmella Evans-Molina 1,3,4,5,6,7,8* Departments of 1Pediatrics, 3Medicine, 4Anatomy, Cell Biology & Physiology, 5Biochemistry & Molecular Biology, the 6Center for Diabetes & Metabolic Diseases, and the 7Herman B. Wells Center for Pediatric Research, Indiana University School of Medicine, Indianapolis, IN 46202; 2Department of BioHealth Informatics, Indiana University-Purdue University Indianapolis, Indianapolis, IN, 46202; 8Roudebush VA Medical Center, Indianapolis, IN 46202. *Corresponding Author(s): Carmella Evans-Molina, MD, PhD ([email protected]) Indiana University School of Medicine, 635 Barnhill Drive, MS 2031A, Indianapolis, IN 46202, Telephone: (317) 274-4145, Fax (317) 274-4107 Running Title: Golgi Stress Response in Diabetes Word Count: 4358 Number of Figures: 6 Keywords: Golgi apparatus stress, Islets, β cell, Type 1 diabetes, Type 2 diabetes 1 Diabetes Publish Ahead of Print, published online August 20, 2020 Diabetes Page 2 of 781 ABSTRACT The Golgi apparatus (GA) is an important site of insulin processing and granule maturation, but whether GA organelle dysfunction and GA stress are present in the diabetic β-cell has not been tested. We utilized an informatics-based approach to develop a transcriptional signature of β-cell GA stress using existing RNA sequencing and microarray datasets generated using human islets from donors with diabetes and islets where type 1(T1D) and type 2 diabetes (T2D) had been modeled ex vivo. To narrow our results to GA-specific genes, we applied a filter set of 1,030 genes accepted as GA associated. -
A Label-Free Cellular Proteomics Approach to Decipher the Antifungal Action of Dimiq, a Potent Indolo[2,3- B]Quinoline Agent, Against Candida Albicans Biofilms
A Label-Free Cellular Proteomics Approach to Decipher the Antifungal Action of DiMIQ, a Potent Indolo[2,3- b]Quinoline Agent, against Candida albicans Biofilms Robert Zarnowski 1,2*, Anna Jaromin 3*, Agnieszka Zagórska 4, Eddie G. Dominguez 1,2, Katarzyna Sidoryk 5, Jerzy Gubernator 3 and David R. Andes 1,2 1 Department of Medicine, School of Medicine & Public Health, University of Wisconsin-Madison, Madison, WI 53706, USA; [email protected] (E.G.D.); [email protected] (D.R.A.) 2 Department of Medical Microbiology, School of Medicine & Public Health, University of Wisconsin-Madison, Madison, WI 53706, USA 3 Department of Lipids and Liposomes, Faculty of Biotechnology, University of Wroclaw, 50-383 Wroclaw, Poland; [email protected] 4 Department of Medicinal Chemistry, Jagiellonian University Medical College, 30-688 Cracow, Poland; [email protected] 5 Department of Pharmacy, Cosmetic Chemicals and Biotechnology, Team of Chemistry, Łukasiewicz Research Network-Industrial Chemistry Institute, 01-793 Warsaw, Poland; [email protected] * Correspondence: [email protected] (R.Z.); [email protected] (A.J.); Tel.: +1-608-265-8578 (R.Z.); +48-71-3756203 (A.J.) Label-Free Cellular Proteomics of Candida albicans biofilms treated with DiMIQ Identified Proteins Accession # Alternate ID Gene names (ORF ) WT DIMIQ Z SCORE Proteins induced by DiMIQ Arginase (EC 3.5.3.1) A0A1D8PP00 CAR1 CAALFM_C504490CA 0.000 6.648 drug induced Glucan 1,3-beta-glucosidase BGL2 (EC 3.2.1.58) (Exo-1Q5AMT2 BGL2 CAALFM_C402250CA -
Overexpression of Superoxide Dismutase 3 Gene Blocks High-Fat Diet-Induced Obesity, Fatty Liver and Insulin Resistance
Gene Therapy (2014) 21, 840–848 © 2014 Macmillan Publishers Limited All rights reserved 0969-7128/14 www.nature.com/gt ORIGINAL ARTICLE Overexpression of superoxide dismutase 3 gene blocks high-fat diet-induced obesity, fatty liver and insulin resistance RCui1,2, M Gao2,SQu1 and D Liu2 Oxidative stress has an important role in the development of obesity and obesity-associated metabolic disorders. As an endogenous antioxidant enzyme, superoxide dismutase 3 (SOD3) has the potential to affect diet-induced obesity and obesity- associated complications. In the current work, we overexpressed SOD3 in C57BL/6 mice fed a high-fat diet (HFD) to study its effect on HFD-induced obesity, fatty liver and insulin resistance. We demonstrated that the Sod3 gene transfer blocked HFD-induced obesity, fatty liver and insulin resistance. Real-time PCR analysis of adipose and liver tissues revealed that overexpression of the Sod3 gene suppressed expression of pro-inflammatory genes in adipose tissue including F4/80, Tnfα, Cd11c, Mcp1 and Il6, and increased expression of anti-inflammatory genes such as adiponectin. In the liver, high levels of SOD3 activity in animals enhanced expression of the genes responsible for energy expenditure including Cpt1α, Cpt1β, Pgc1α, Pgc1β and Ucp2. These results suggest that overexpression of the Sod3 gene through gene transfer is an effective approach in preventing diet-induced obesity and obesity-associated complications. Gene Therapy (2014) 21, 840–848; doi:10.1038/gt.2014.64; published online 17 July 2014 INTRODUCTION hydrodynamic Sod3 gene transfer blocked HFD-induced weight Obesity is a causative factor in the development of several gain, insulin resistance and alleviated fatty liver. -
The Thyroid Hormone Mediation
Sabatino et al. Vessel Plus 2021;5:3 Vessel Plus DOI: 10.20517/2574-1209.2020.62 Review Open Access Oxidative stress and heart disease: the thyroid hormone mediation Laura Sabatino1, Rudina Ndreu1, Cristina Vassalle2 1National Research Council, Institute of Clinical Physiology, CNR, Pisa 56124, Italy. 2Fondazione CNR-Regione Toscana Gabriele Monasterio, Pisa 56124, Italy. Correspondence to: Dr. Laura Sabatino, Institute of Clinical Physiology, CNR, Via Moruzzi 1, Pisa 56124, Italy. E-mail: [email protected] How to cite this article: Sabatino L, Ndreu R, Vassalle C. Oxidative stress and heart disease: the thyroid hormone mediation. Vessel Plus 2021;5:3. http://dx.doi.org/10.20517/2574-1209.2020.62. Received: 30 Oct 2020 First Decision: 30 Nov 2020 Revised: 5 Dec 2020 Accepted: 16 Dec 2020 Published: 15 Jan 2021 Academic Editor: Sampath Parthasarathy, Narasimham L. Parinandi Copy Editor: Miao Zhang Production Editor: Jing Yu Abstract Received: First Decision: Revised: Accepted: Published: x The heart is one of the principal targets of thyroid hormones (TH) action, affecting cardiac contractility, heart rate, and diastolic function. Oxidative damage is pivotal for onset and development of cardiovascular disease (CVD) and Science Editor: Copy Editor: Production Editor: Jing Yu heart failure. Specifically, free radical generation is associated to hyper-metabolic state in hyperthyroidism, whereas hypo-metabolic state induced by hypothyroidism leads to a decrease of oxidative stress. In the present review, the role of oxidative damage in CVD and failing heart-TH interplay will be considered. The main oxidative events leading to cardiac dysfunction and TH cardiac regulation at genomic and non-genomic levels will be discussed, as well as role of TH in cardioprotection and reversion of cardiac remodeling. -
Extracellular Superoxide Dismutase Protects Against Pulmonary Emphysema by Attenuating Oxidative Fragmentation of ECM
Extracellular superoxide dismutase protects against pulmonary emphysema by attenuating oxidative fragmentation of ECM Hongwei Yaoa, Gnanapragasam Arunachalama, Jae-woong Hwanga, Sangwoon Chunga, Isaac K. Sundara, Vuokko L. Kinnulab, James D. Crapoc, and Irfan Rahmana,1 aDepartment of Environmental Medicine, Lung Biology and Disease Program, University of Rochester Medical Center, Rochester, NY 14642; bPulmonary Division, Department of Medicine, University of Helsinki and Helsinki University Hospital, FIN-00029 Helsinki, Finland; and cDepartment of Medicine, National Jewish Medical and Research Center, Denver, CO 80206 Edited by Irwin Fridovich, Duke University Medical Center, Durham, NC, and approved July 27, 2010 (received for review June 3, 2010) Extracellular superoxide dismutase (ECSOD or SOD3) is highly celerated decline in lung function. In addition to being a direct • ─ expressed in lungs and functions as a scavenger of O2 . ECM exogenous source of reactive oxygen species (ROS), CS also fragmentation, which can be triggered by oxidative stress, partic- induces endogenous production of ROS from activated inflam- ipates in the pathogenesis of chronic obstructive pulmonary dis- matory cells in the lung. ROS can fragment ECM components, such ease (COPD) through attracting inflammatory cells into the lungs. as elastin, heparan sulfate, and hyaluronan, which is an important The level of SOD3 is significantly decreased in lungs of patients factor in triggering lung inflammation and causing subsequent with COPD. However, the role of endogenous SOD3 in the devel- airspace enlargement (15–18). SOD3 has been shown to bind to opment/progression of emphysema is unknown. We hypothesized ECM through its positively charged C-terminal, thereby preventing that SOD3 protects against emphysema by attenuating oxidative oxidative fragmentation of ECM components (15, 19). -
The Emerging Roles of Antioxidant Enzymes by Dietary Phytochemicals in Vascular Diseases
life Review The Emerging Roles of Antioxidant Enzymes by Dietary Phytochemicals in Vascular Diseases Seung Eun Lee and Yong Seek Park * Department of Microbiology, School of Medicine, Kyung Hee University, Seoul 02447, Korea; [email protected] * Correspondence: [email protected]; Tel.: +82-02-961-0267 Abstract: Vascular diseases are major causes of death worldwide, causing pathologies including diabetes, atherosclerosis, and chronic obstructive pulmonary disease (COPD). Exposure of the vascular system to a variety of stressors and inducers has been implicated in the development of various human diseases, including chronic inflammatory diseases. In the vascular wall, antioxidant enzymes form the first line of defense against oxidative stress. Recently, extensive research into the beneficial effects of phytochemicals has been conducted; phytochemicals are found in commonly used spices, fruits, and herbs, and are used to prevent various pathologic conditions, including vascular diseases. The present review aims to highlight the effects of dietary phytochemicals role on antioxidant enzymes in vascular diseases. Keywords: vascular diseases; antioxidant enzymes; bioactive compounds 1. Introduction Citation: Lee, S.E.; Park, Y.S. The Vascular diseases are responsible for numerous deaths annually worldwide. The Emerging Roles of Antioxidant pathogenesis of vascular disease involves the activation of pro-inflammatory signaling Enzymes by Dietary Phytochemicals pathways, expression of cytokines/chemokines, and elevated oxidative stress. Exposure in Vascular Diseases. Life 2021, 11, 199. https://doi.org/10.3390/ to oxidative stress may directly injure the vasculature and induce vascular dysfunction life11030199 by producing dysregulation of the immune response. Oxidative stress is caused by an imbalance between the production and accumulation of reactive oxygen species (ROS) and Academic Editor: Payaningal the capacity of antioxidant defense mechanisms favoring oxidants [1]. -
Association of Genetic Polymorphisms in SOD2, SOD3, GPX3, and GSTT1
Association of genetic polymorphisms in SOD2, SOD3, GPX3, and GSTT1 with hypertriglyceridemia and low HDL-C level in subjects with high risk of coronary artery disease Nisa Decharatchakul1,2, Chatri Settasatian2,3, Nongnuch Settasatian2,4, Nantarat Komanasin2,4, Upa Kukongviriyapan2,5, Phongsak Intharaphet2,6 and Vichai Senthong2,6,7 1 Biomedical Sciences Program, Graduate School, Khon Kaen University, Khon Kaen, Thailand 2 Cardiovascular Research Group, Khon Kaen University, Khon Kaen, Thailand 3 Department of Pathology, Faculty of Medicine, Khon Kaen University, Khon Kaen, Thailand 4 School of Medical Technology, Faculty of Associated Medical Sciences, Khon Kaen University, Khon Kaen, Thailand 5 Department of Physiology, Faculty of Medicine, Khon Kaen University, Khon Kaen, Thailand 6 Queen Sirikit Heart Center of the Northeast, Khon Kaen University, Khon Kaen, Thailand 7 Department of Internal Medicine, Faculty of Medicine, Khon Kaen University, Khon Kaen, Thailand ABSTRACT Background. Oxidative stress modulates insulin resistant-related atherogenic dys- lipidemia: hypertriglyceridemia (HTG) and low high-density lipoprotein cholesterol (HDL-C) level. Gene polymorphisms in superoxide dismutase (SOD2 and SOD3), glutathione peroxidase-3 (GPX3), and glutathione S-transferase theta-1 (GSTT1) may enable oxidative stress-related lipid abnormalities and severity of coronary atherosclerosis. The present study investigated the associations of antioxidant-related gene polymorphisms with atherogenic dyslipidemia and atherosclerotic severity in subjects with high risk of coronary artery disease (CAD). Submitted 13 April 2019 Methods. Study population comprises of 396 subjects with high risk of CAD. Gene Accepted 4 July 2019 Published 1 August 2019 polymorphisms: SOD2 rs4880, SOD3 rs2536512 and rs2855262, GPX rs3828599, and GSTT1 (deletion) were evaluated the associations with HTG, low HDL-C, high Corresponding author Chatri Settasatian, [email protected], TG/HDL-C ratio, and severity of coronary atherosclerosis. -
Novel and Converging Ways of NOX2 and SOD3 in Trafficking and Redox
antioxidants Review Novel and Converging Ways of NOX2 and SOD3 in Trafficking and Redox Signaling in Macrophages Steen Vang Petersen 1 , Nanna Bach Poulsen 2, Cecilie Linneberg Matthiesen 1 and Frederik Vilhardt 2,* 1 Department of Biomedicine, Aarhus University, 8000 Aarhus C, Denmark; [email protected] (S.V.P.); [email protected] (C.L.M.) 2 Department of Cellular and Molecular Medicine, Copenhagen University, 2200 Copenhagen, Denmark; [email protected] * Correspondence: [email protected]; Tel.: +45-35327120 Abstract: Macrophages and related tissue macrophage populations use the classical NADPH oxidase (NOX2) for the regulated production of superoxide and derived oxidants for pathogen combat and redox signaling. With an emphasis on macrophages, we discuss how sorting into secretory storage vesicles, agonist-responsive membrane trafficking, and segregation into sphingolipid and cholesterol-enriched microdomains (lipid rafts) determine the subcellular distribution and spatial organization of NOX2 and superoxide dismutase-3 (SOD3). We discuss how inflammatory activation of macrophages, in part through small GTPase Rab27A/B regulation of the secretory compartments, mediates the coalescence of these two proteins on the cell surface to deliver a focalized hydrogen peroxide output. In interplay with membrane-embedded oxidant transporters and redox sensitive target proteins, this arrangement allows for the autocrine and paracrine signaling, which govern macrophage activation states and transcriptional programs. By discussing examples of autocrine and paracrine redox signaling, we highlight why formation of spatiotemporal microenvironments Citation: Petersen, S.V.; Poulsen, N.B.; Linneberg Matthiesen, C.; where produced superoxide is rapidly converted to hydrogen peroxide and conveyed immediately Vilhardt, F. Novel and Converging to reach redox targets in proximal vicinity is required for efficient redox signaling. -
A Small Molecule Promotes Cartilage Extracellular Matrix Generation and Inhibits Osteoarthritis Development
ARTICLE https://doi.org/10.1038/s41467-019-09839-x OPEN A small molecule promotes cartilage extracellular matrix generation and inhibits osteoarthritis development Yuanyuan Shi1,2, Xiaoqing Hu1,2, Jin Cheng1, Xin Zhang1, Fengyuan Zhao1, Weili Shi1, Bo Ren1, Huilei Yu1, Peng Yang1, Zong Li1, Qiang Liu1, Zhenlong Liu 1, Xiaoning Duan1, Xin Fu1, Jiying Zhang1, Jianquan Wang1 & Yingfang Ao1 1234567890():,; Degradation of extracellular matrix (ECM) underlies loss of cartilage tissue in osteoarthritis, a common disease for which no effective disease-modifying therapy currently exists. Here we describe BNTA, a small molecule with ECM modulatory properties. BNTA promotes gen- eration of ECM components in cultured chondrocytes isolated from individuals with osteoarthritis. In human osteoarthritic cartilage explants, BNTA treatment stimulates expression of ECM components while suppressing inflammatory mediators. Intra-articular injection of BNTA delays the disease progression in a trauma-induced rat model of osteoarthritis. Furthermore, we identify superoxide dismutase 3 (SOD3) as a mediator of BNTA activity. BNTA induces SOD3 expression and superoxide anion elimination in osteoarthritic chondrocyte culture, and ectopic SOD3 expression recapitulates the effect of BNTA on ECM biosynthesis. These observations identify SOD3 as a relevant drug target, and BNTA as a potential therapeutic agent in osteoarthritis. 1 Institute of Sports Medicine, Beijing Key Laboratory of Sports Injuries, Peking University Third Hospital, 100191 Beijing, China. 2These authors contributed equally: Yuanyuan Shi, Xiaoqing Hu. Correspondence and requests for materials should be addressed to J.W. (email: [email protected]) or to Y.A. (email: [email protected]) NATURE COMMUNICATIONS | (2019) 10:1914 | https://doi.org/10.1038/s41467-019-09839-x | www.nature.com/naturecommunications 1 ARTICLE NATURE COMMUNICATIONS | https://doi.org/10.1038/s41467-019-09839-x steoarthritis (OA) is the most prevalent musculoskeletal compared with vehicle (Fig. -
The Dynamic Uptake and Release of SOD3 from Intracellular Stores in Macrophages Modulates the Inflammatory Response
The dynamic uptake and release of SOD3 from intracellular stores in macrophages modulates the inflammatory response Hu, Lili; Zachariae, Elias D.; Larsen, Ulrike G.; Vilhardt, Frederik; Petersen, Steen V. Published in: Redox Biology DOI: 10.1016/j.redox.2019.101268 Publication date: 2019 Document version Publisher's PDF, also known as Version of record Document license: CC BY Citation for published version (APA): Hu, L., Zachariae, E. D., Larsen, U. G., Vilhardt, F., & Petersen, S. V. (2019). The dynamic uptake and release of SOD3 from intracellular stores in macrophages modulates the inflammatory response. Redox Biology, 26, [101268]. https://doi.org/10.1016/j.redox.2019.101268 Download date: 25. Sep. 2021 Redox Biology 26 (2019) 101268 Contents lists available at ScienceDirect Redox Biology journal homepage: www.elsevier.com/locate/redox The dynamic uptake and release of SOD3 from intracellular stores in macrophages modulates the inflammatory response T Lili Hua, Elias D. Zachariaea, Ulrike G. Larsena, Frederik Vilhardtb, Steen V. Petersena,* a Department of Biomedicine, Aarhus University, DK-8000, Aarhus C, Denmark b Department of Cellular and Molecular Medicine, University of Copenhagen, DK-2200, Copenhagen N, Denmark ARTICLE INFO ABSTRACT Keywords: Superoxide dismutase 3 (SOD3) is an extracellular enzyme with the capacity to modulate extracellular redox Superoxide dismutase 3 (SOD3) conditions by catalyzing the dismutation of superoxide to hydrogen peroxide. In addition to synthesis and re- Macrophage lease of this extracellular protein via the secretory pathway, several studies have shown that the protein also Internalization localizes to intracellular compartments in neutrophils and macrophages. Here we show that human macrophages Secretion release SOD3 from an intracellular compartment within 30 min following LPS stimulation. -
Supplementary Materials: Molecular Signature of Subtypes of Non- Small Cell Lung Cancer by Large-Scale Transcriptional Profiling
Cancers 2020 S1 of S18 Supplementary Materials: Molecular Signature of Subtypes of Non- Small Cell Lung Cancer by Large-Scale Transcriptional Profiling: Identification of Key Modules and Genes by Weighted Gene Co- Expression Network Analysis (WGCNA) Magdalena Niemira, Francois Collin, Anna Szalkowska, Agnieszka Bielska, Karolina Chwialkowska, Joanna Reszec, Jacek Niklinski, Miroslaw Kwasniewski and Adam Kretowski Cancers 2020 S2 of S18 A B Figure S1. The top-ranked enriched canonical pathway identified in (A) SCC and (B) ADC using IPA: Eicosanoid signalling pathway. Cancers 2020 S3 of S18 A Cancers 2020 S4 of S18 Figure S2. The second-ranked enriched canonical pathway identified in (A) SCC and (B) ADC using IPA: Agranulocyte adhesion and diapedesis. Cancers 2020 S5 of S18 Figure S3. The top-ranked enriched canonical pathway identified only in lung ADC: MIF regulation of innate immunity. A B Figure S4. Cluster dendograms of the gene clusters of (A) LUAD and (B) LUSC subset from TCGA database. Cancers 2020 S6 of S18 A B C D Figure S5. Protein-protein interaction (PPI) network of genes in the red (A), lightcyan (B), darkorange (C), yellow (D) modules in ADC. The networks were constructed using Cytoscape v. 3.7.2. software. Cancers 2020 S7 of S18 A B Figure S6. Protein-protein interaction (PPI) network of genes in the blue (A) and (B) modules in SCC. The networks were constructed using Cytoscape v. 3.7.2. software. Cancers 2020 S8 of S18 Table S1. Upstream regulator analysis of DEGs in lung SCC predicted by IPA. Upstream Prediction Target -
An Insight Into the Role of Extracellular Vesicles-Mediated Oxidative Stress Responses Chontida Yarana University of Kentucky, [email protected]
University of Kentucky UKnowledge Toxicology and Cancer Biology Faculty Toxicology and Cancer Biology Publications 9-28-2017 Chemotherapy-Induced Tissue Injury: An Insight into the Role of Extracellular Vesicles-Mediated Oxidative Stress Responses Chontida Yarana University of Kentucky, [email protected] Daret K. St. Clair University of Kentucky, [email protected] Right click to open a feedback form in a new tab to let us know how this document benefits oy u. Follow this and additional works at: https://uknowledge.uky.edu/toxicology_facpub Part of the Medical Toxicology Commons Repository Citation Yarana, Chontida and St. Clair, Daret K., "Chemotherapy-Induced Tissue Injury: An Insight into the Role of Extracellular Vesicles- Mediated Oxidative Stress Responses" (2017). Toxicology and Cancer Biology Faculty Publications. 62. https://uknowledge.uky.edu/toxicology_facpub/62 This Review is brought to you for free and open access by the Toxicology and Cancer Biology at UKnowledge. It has been accepted for inclusion in Toxicology and Cancer Biology Faculty Publications by an authorized administrator of UKnowledge. For more information, please contact [email protected]. Chemotherapy-Induced Tissue Injury: An Insight into the Role of Extracellular Vesicles-Mediated Oxidative Stress Responses Notes/Citation Information Published in Antioxidants, v. 6, issue 4, 75, p. 1-17. © 2017 by the authors. Licensee MDPI, Basel, Switzerland. This article is an open access article distributed under the terms and conditions of the Creative Commons Attribution (CC BY) license (http://creativecommons.org/licenses/by/4.0/). Digital Object Identifier (DOI) https://doi.org/10.3390/antiox6040075 This review is available at UKnowledge: https://uknowledge.uky.edu/toxicology_facpub/62 antioxidants Review Chemotherapy-Induced Tissue Injury: An Insight into the Role of Extracellular Vesicles-Mediated Oxidative Stress Responses Chontida Yarana 1,2 and Daret K.