Ion Channels in Drug Discovery
Total Page:16
File Type:pdf, Size:1020Kb
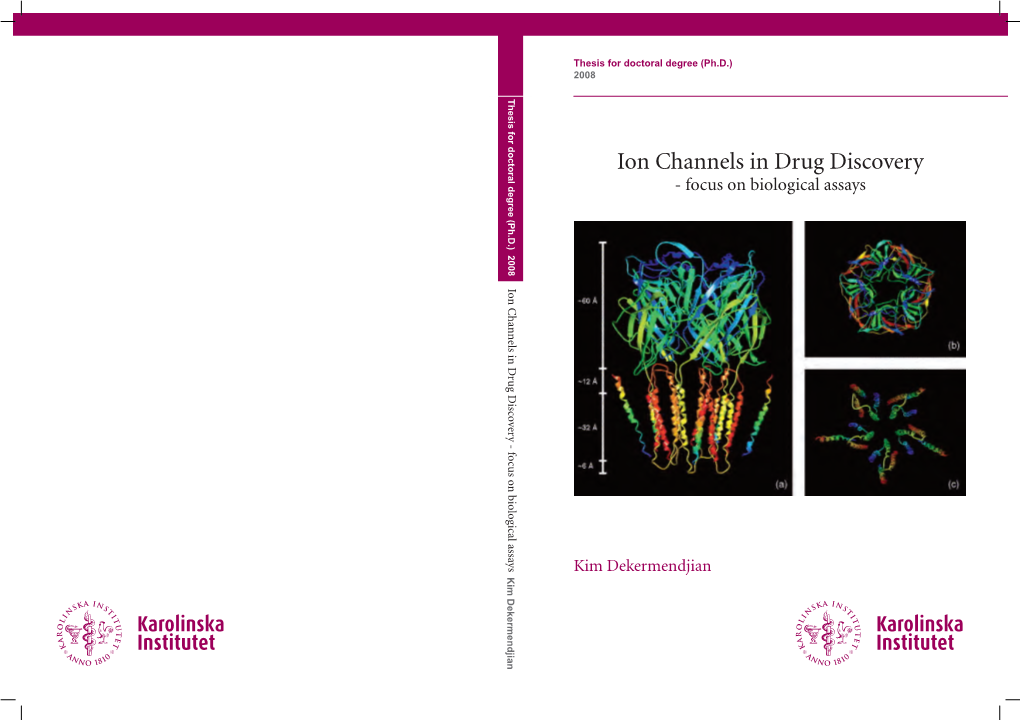
Load more
Recommended publications
-
Exploring Non-Covalent Interactions Between Drug-Like Molecules and the Protein Acetylcholinesterase
Exploring non-covalent interactions between drug-like molecules and the protein acetylcholinesterase Lotta Berg Doctoral Thesis, 2017 Department of Chemistry Umeå University, Sweden This work is protected by the Swedish Copyright Legislation (Act 1960:729) ISBN: 978-91-7601-644-2 Elektronisk version tillgänglig på http://umu.diva-portal.org/ Printed by: VMC-KBC Umeå Umeå, Sweden, 2017 “Life is a relationship between molecules, not a property of any one molecule” Emile Zuckerkandl and Linus Pauling 1962 i Table of contents Abstract vi Sammanfattning vii Papers covered in the thesis viii List of abbreviations x 1. Introduction 1 1.1. Drug discovery 1 1.2. The importance of non-covalent interactions 1 1.3. Fundamental forces of non-covalent interactions 2 1.4. Classes of non-covalent interactions 3 1.5. Hydrogen bonds 3 1.5.1. Definition of the hydrogen bond 3 1.5.2. Classical and non-classical hydrogen bonds 4 1.5.3. CH∙∙∙Y hydrogen bonds 5 1.6. Interactions between aromatic rings 6 1.7. Thermodynamics of binding 7 1.8. The essential enzyme acetylcholinesterase 8 1.8.1. The relevance of research focused on AChE 8 1.8.2. The structure of AChE 9 1.8.3. AChE in drug discovery 10 2. Scope of the thesis 12 3. Background to techniques and methods 13 3.1. Structure determination by X-ray crystallography 13 3.1.1. The significance of crystal structures of proteins 13 3.1.2. Data collection and structure refinement 13 3.1.3. Electron density maps 14 3.1.4. -
Clinical Pharmacology of Antiмcancer Agents
A CLINI CLINICAL PHARMACOLOGY OF NTI- C ANTI-CANCER AGENTS C AL PHARMA AN Cristiana Sessa, Luca Gianni, Marina Garassino, Henk van Halteren www.esmo.org C ER A This is a user-friendly handbook, designed for young GENTS medical oncologists, where they can access the essential C information they need for providing the treatment which could OLOGY OF have the highest chance of being effective and tolerable. By finding out more about pharmacology from this handbook, young medical oncologists will be better able to assess the different options available and become more knowledgeable in the evaluation of new treatments. ESMO ESMO Handbook Series European Society for Medical Oncology CLINICAL PHARMACOLOGY OF Via Luigi Taddei 4, 6962 Viganello-Lugano, Switzerland Handbook Series ANTI-CANCER AGENTS Cristiana Sessa, Luca Gianni, Marina Garassino, Henk van Halteren ESMO Press · ISBN 978-88-906359-1-5 ISBN 978-88-906359-1-5 www.esmo.org 9 788890 635915 ESMO Handbook Series handbook_print_NEW.indd 1 30/08/12 16:32 ESMO HANDBOOK OF CLINICAL PHARMACOLOGY OF ANTI-CANCER AGENTS CM15 ESMO Handbook 2012 V14.indd1 1 2/9/12 17:17:35 ESMO HANDBOOK OF CLINICAL PHARMACOLOGY OF ANTI-CANCER AGENTS Edited by Cristiana Sessa Oncology Institute of Southern Switzerland, Bellinzona, Switzerland; Ospedale San Raffaele, IRCCS, Unit of New Drugs and Innovative Therapies, Department of Medical Oncology, Milan, Italy Luca Gianni Ospedale San Raffaele, IRCCS, Unit of New Drugs and Innovative Therapies, Department of Medical Oncology, Milan, Italy Marina Garassino Oncology Department, Istituto Nazionale dei Tumori, Milan, Italy Henk van Halteren Department of Internal Medicine, Gelderse Vallei Hospital, Ede, The Netherlands ESMO Press CM15 ESMO Handbook 2012 V14.indd3 3 2/9/12 17:17:35 First published in 2012 by ESMO Press © 2012 European Society for Medical Oncology All rights reserved. -
Biological Target and Its Mechanism He Zuan* Department of Pharmacology, School of Medicine,Shenzhen University, Shenzhen 518000, China
OPEN ACCESS Freely available online Journal of Cell Signaling Editorial Biological Target and Its Mechanism He Zuan* Department of Pharmacology, School of Medicine,Shenzhen University, Shenzhen 518000, China EDITORIAL NOTE 1. There is no direct change in the biological target, but the binding of the substance stops other endogenous substances (such Biological target is whatsoever within an alive organism to which as triggering hormones) from binding to the target. Contingent some other entity (like an endogenous drug or ligand) is absorbed on the nature of the target, this effect is mentioned as receptor and binds, subsequent in a modification in its function or antagonism, ion channel blockade or enzyme inhibition. behaviour. For examples of mutual biological targets are nucleic acids and proteins. Definition is context dependent, and can refer 2. A conformational change in the target is induced by the stimulus to the biological target of a pharmacologically active drug multiple, which results in a change in target function. This change in function the receptor target of a hormone like insulin, or some other target can mimic the effect of the endogenous substance in which case of an external stimulus. Biological targets are most commonly the effect is referred to as receptor agonism or be the opposite of proteins such as ion channels, enzymes, and receptors. The term the endogenous substance which in the case of receptors is referred "biological target" is regularly used in research of pharmaceutical to as inverse agonism. to describe the nastive protein in body whose activity is altered Conservation Ecology: These biological targets are conserved by a drug subsequent in a specific effect, which may a desirable across species, making pharmaceutical pollution of the therapeutic effect or an unwanted adverse effect. -
Uncovering New Drug Properties in Target-Based Drug-Drug Similarity Networks
bioRxiv preprint doi: https://doi.org/10.1101/2020.03.12.988600; this version posted March 12, 2020. The copyright holder for this preprint (which was not certified by peer review) is the author/funder, who has granted bioRxiv a license to display the preprint in perpetuity. It is made available under aCC-BY 4.0 International license. Uncovering new drug properties in target-based drug-drug similarity networks Lucret¸ia Udrescu1, Paul Bogdan2, Aim´eeChi¸s3, Ioan Ovidiu S^ırbu3,6, Alexandru Top^ırceanu4, Renata-Maria V˘arut¸5, Mihai Udrescu4,6* 1 Department of Drug Analysis, "Victor Babe¸s",University of Medicine and Pharmacy Timi¸soara,Timi¸soara,Romania 2 Ming Hsieh Department of Electrical Engineering, University of Southern California, Los Angeles, CA, USA 3 Department of Biochemistry, "Victor Babe¸s"University of Medicine and Pharmacy Timi¸soara,Timi¸soara,Romania 4 Department of Computer and Information Technology, Politehnica University of Timi¸soara,Timi¸soara,Romania 5 Faculty of Pharmacy, University of Medicine and Pharmacy of Craiova, Craiova, Romania 6 Timi¸soaraInstitute of Complex Systems, Timi¸soara,Romania * [email protected] Abstract Despite recent advances in bioinformatics, systems biology, and machine learning, the accurate prediction of drug properties remains an open problem. Indeed, because the biological environment is a complex system, the traditional approach { based on knowledge about the chemical structures { cannot fully explain the nature of interactions between drugs and biological targets. Consequently, in this paper, we propose an unsupervised machine learning approach that uses the information we know about drug-target interactions to infer drug properties. -
MDMA, MBDB' and I! I the Classichallucinogens
Differences Between the Mechanism I t of Action of MDMA, MBDB' and i! I the ClassicHallucinogens. i Identification of a New Therapeutic Class: Entactogens Jawi'ri", ! 7 U$ DAVID E. NICHOLS, PH.D.* The widespread use of psychedelic drugs, such as quantitative structure-activity relationships (QSARs). lysergic ac;.d diethylamide (LSD), during the 1960's and Simply stated, attempts are made to find quantitative 1970's IcC to severe reactions by governmental agencies correlations (or equations) that relate biological activity to and proscriptions against their use. However, with the fundamental properties of the molecule. This approach is high deg _e of interest in mind-altering drugs in the being widely developed in the pharmaceutical industry in United States, as evidenced by their widespread popular- order to understand more fully how particular types of ity, it wasonlyamatteroftimebeforenewdrugsappeared drugs work, and to be able to predict which additional that were developed outside of the pharmaceutical corn- molecules should be synthesized. It should also be added panics, that no equations have been developed that adequately j Nearly 70 years after its first synthesis, 3,4- correlate hallucinogenic or psychedelic activity with any methy'enedioxymethamphetamine (MDMA) was redis- particular molecular property. covered. Although it had its' more recent origin in the class In this context, it is important to define clearly the of dr. gs that is generally defined as psychedelic or hallu- type of biological activity that is being measured. It is cino enic, it clearly appears different from LSD. In hu- typical that if a molecule has several different sites of mar , MDMA induces a state of reduced anxiety and action in the brain, that each oneof these actions may be lc .red defensiveness that makes it attractive to thera- related to entirely different structural features or proper- pi . -
Full Text (PDF)
Review TheProgressandPromiseofMolecularImagingProbesin Oncologic Drug Development Gary J. Kelloff,1Kenneth A. Krohn,3 Steven M. Larson,4 Ralph Weissleder,5 David A. Mankoff,3 John M. Hoffman,1Jeanne M. Link, 3 Kathryn Z. Guyton,6 William C. Eckelman,2 Howard I. Scher,4 Joyce O’Shaughnessy,7 Bruce D. Cheson,8 Caroline C. Sigman,6 James L. Tatum,1 George Q. Mills,9 Daniel C. Sullivan,1and Janet Woodcock10 Abstract As addressed by the recent Food and Drug Administration Critical Path Initiative, tools are urgently needed to increase the speed, efficiency, and cost-effectiveness of drug development for cancer and other diseases. Molecular imaging probes developed based on recent scientific advances have great potential as oncologic drug development tools. Basic science studies using molecular imaging probes can help to identify and characterize disease-specific targets for oncologic drug therapy. Imaging end points, based on these disease-specific biomarkers, hold great promise to better define, stratify, and enrich study groups and to provide direct biological measures of response. Imaging-based biomarkers also have promise for speeding drug evaluation by supplementing or replacing preclinical and clinical pharmacokinetic and pharmacodynamic evaluations, including target interaction and modulation. Such analyses may be particularly valuable in early comparative studies among candidates designed to interact with the same molecular target. Finally, as response biomarkers, imaging end points that characterize tumor vitality, growth, or apoptosis can also serve as early surrogates of therapy success. This article outlines the scientific basis of oncology imaging probes and presents examples of probes that could facilitate progress. The current regulatory opportunities for new and existing probe development and testing are also reviewed, with a focus on recent Food and Drug Administra- tion guidance to facilitate early clinical development of promising probes. -
Next Generation Risk Assessment of Human Exposure to Anti-Androgens Using Newly Defined Comparator Compound Values
Toxicology in Vitro 73 (2021) 105132 Contents lists available at ScienceDirect Toxicology in Vitro journal homepage: www.elsevier.com/locate/toxinvit Next generation risk assessment of human exposure to anti-androgens using newly defined comparator compound values Tessa C.A. van Tongeren a,*, Thomas E. Moxon b, Matthew P. Dent b, Hequn Li b, Paul L. Carmichael b, Ivonne M.C.M. Rietjens a a Division of Toxicology, Wageningen University and Research, 6700, EA, Wageningen, the Netherlands b Unilever Safety and Environmental Assurance Centre, Colworth Science Park, Sharnbrook, Bedfordshire MK44 1LQ, UK ARTICLE INFO ABSTRACT Keywords: Next Generation Risk Assessment (NGRA) can use the so-called Dietary Comparator Ratio (DCR) to evaluate the Risk assessment safety of a definedexposure to a compound of interest. The DCR compares the Exposure Activity Ratio (EAR) for 3R compliant method the compound of interest, to the EAR of an established safe level of human exposure to a comparator compound Androgen receptor with the same putative mode of action. A DCR ≤ 1 indicates the exposure evaluated is safe. The present study Dietary comparator aimed at defining adequate and safe comparator compound exposures for evaluation of anti-androgenic effects, In vitro/in silico approaches using 3,3-diindolylmethane (DIM), from cruciferous vegetables, and the anti-androgenic drug bicalutamide (BIC). EAR values for these comparator compounds were definedusing the AR-CALUX assay. The adequacy of the new comparator EAR values was evaluated using PBK modelling and by comparing the generated DCRs of a series of test compound exposures to actual knowledge on their safety regarding in vivo anti-androgenicity. -
Using in Vitro Toxcast Assays to Evaluate Mechanistic Plausibility
Using In vitro ToxCast Assays to Evaluate Mechanistic Plausibility and Build Confidence in the Selection of Analogues for Quantitative Read-Across: A Case Study on p,p’-Dichlorodiphenyldichloroethane Lucina E. Lizarraga, Jeffry L. Dean, J. Phillip Kaiser, Scott C. Wesselkamper, Jason C. Lambert, Elizabeth O. Owens, Belinda Hawkins, Q. Jay Zhao. National Center for Environmental Assessment (NCEA), U.S. Environmental Protection Agency, Cincinnati, Ohio 45268, USA Lucina E. Lizarraga l [email protected] l 513-487 2648 Overview Bioactivity Similarity Comparisons Evaluating Mechanistic Plausibility for Liver and Reproductive Toxicity • Deriving human health reference values for environmental chemicals has traditionally relied on toxicity data from humans and/or experimental animals p,p’-DDD and Analogues Exhibit Similarities in Cell-specific Responses and Target Gene p,p’-DDD and Analogues Exhibit Similar Upregulation of Steroid/Xenobiotic-sensing Pathways in In Vitro ToxCast Assays Conducted in Human Liver Cells Nuclear Receptors in In Vitro ToxCast Assays Conducted in Hepatoma HepG2 Cells • In the absence of in vivo toxicity data, new approach methodologies such as read-across can be used to fill data gaps for a target chemical using known information from a source analogue Figure 2. Bioactivity data for p,p’-DDD and Analogues in ToxCast Assays Conducted in • A read-across approach illustrated below (Figure 1) was applied to assist in screening-level Human Hepatoma HepG2 Cells and Primary assessment of noncancer oral toxicity for the target, p,p’-DDD, a data-poor chemical known to occur Human Hepatocytes. Scatterplots show AC50 and at contaminated sites in the U.S. scaled activity values for p,p’-DDD, p,p’-DDT, p,p’- DDE and methoxychlor from in vitro assays visualized according to the type of biological response or biological target. -
'Bath Salts' Constituents MDPV, Mephedrone, and Methylone Serena Allen East Tennessee State University
East Tennessee State University Digital Commons @ East Tennessee State University Electronic Theses and Dissertations Student Works 5-2018 The ombinedC Neuropharmacology and Toxicology of Major 'Bath Salts' Constituents MDPV, Mephedrone, and Methylone Serena Allen East Tennessee State University Follow this and additional works at: https://dc.etsu.edu/etd Part of the Medicinal Chemistry and Pharmaceutics Commons, Neuroscience and Neurobiology Commons, Pharmacology Commons, Pharmacy and Pharmaceutical Sciences Commons, and the Toxicology Commons Recommended Citation Allen, Serena, "The ombC ined Neuropharmacology and Toxicology of Major 'Bath Salts' Constituents MDPV, Mephedrone, and Methylone" (2018). Electronic Theses and Dissertations. Paper 3431. https://dc.etsu.edu/etd/3431 This Dissertation - Open Access is brought to you for free and open access by the Student Works at Digital Commons @ East Tennessee State University. It has been accepted for inclusion in Electronic Theses and Dissertations by an authorized administrator of Digital Commons @ East Tennessee State University. For more information, please contact [email protected]. The Combined Neuropharmacology and Toxicology of Major ‘Bath Salts’ Constituents MDPV, Mephedrone, and Methylone A dissertation presented to the faculty of the Department of Biomedical Sciences East Tennessee State University In partial fulfillment of the requirements for the degree Doctor of Philosophy in Biomedical Sciences, Pharmaceutical Science Concentration by Serena A. Allen May 2018 Dr. Brooks Pond, -
Developments in Prostate Cancer Therapy: from the Androgen Receptor to Antiandrogens and Beyond
FARMACIA, 2021, Vol. 69, 3 https://doi.org/10.31925/farmacia.2021.3.1 REVIEW DEVELOPMENTS IN PROSTATE CANCER THERAPY: FROM THE ANDROGEN RECEPTOR TO ANTIANDROGENS AND BEYOND IULIAN PRUTIANU 1, IRINA DRAGA CĂRUNTU 1*, MARIANA BIANCA MANOLE 1, SIMONA ELIZA GIUȘCĂ 1, BIANCA PROFIRE 1, ANDREI DANIEL TIMOFTE 1, LENUȚA PROFIRE 2, BOGDAN GAFTON 3 1Department Morpho-Functional Sciences I - Histology, Pathology, “Grigore T. Popa” University of Medicine and Pharmacy, Iași, Romania 2Department Pharmaceutical Sciences I - Pharmaceutical Chemistry, “Grigore T. Popa” University of Medicine and Pharmacy, Iași, Romania 3Department Medical III - Medical Oncology - Radiotherapy, “Grigore T. Popa” University of Medicine and Pharmacy, Iași, Romania *corresponding author: [email protected] Manuscript received: July 2020 Abstract Prostate cancer is the second most common malignancy in men. The androgenic receptor (AR) is the main therapeutic target for this type of cancer, hormone therapy being the cornerstone of patient management, despite resistance developing over time in some cases. The scientific concern and practical necessity for more effective therapies have led to the introduction of novel drug classes, including new taxanes, PARP inhibitors and immunotherapeutic agents. Studies regarding the complex mechanisms of action which account for the therapeutic effects of these drugs are supported by clinical trials which confirm the optimization of survival parameters. The present article summarizes current diagnostic and therapeutic trends in prostate cancer treatment; it is organized in distinct sections aimed at: (i) the evolution of different concepts and approaches in the histopathological classification, (ii) the structure and function of the androgenic receptor and its truncated variant 7, considered a clear indicator of the lack of response to hormone treatment, (iii) the therapeutic principles in both localized and locally advanced prostate cancer, (iv) the innovative strategies in treating castration-resistant and metastatic prostate cancer. -
Uncovering New Drug Properties in Target-Based Drug-Drug Similarity Networks
bioRxiv preprint doi: https://doi.org/10.1101/2020.03.12.988600; this version posted June 27, 2020. The copyright holder for this preprint (which was not certified by peer review) is the author/funder, who has granted bioRxiv a license to display the preprint in perpetuity. It is made available under aCC-BY 4.0 International license. Uncovering new drug properties in target-based drug-drug similarity networks Lucret¸iaUdrescu1, Paul Bogdan2, Aimee´ Chis¸ 3, Ioan Ovidiu Sˆırbu3,6, Alexandru Topˆırceanu4, Renata-Maria Varut¸˘ 5, and Mihai Udrescu4,6,* 1”Victor Babes¸” University of Medicine and Pharmacy Timis¸oara, Department of Drug Analysis, Timis¸oara 300041, Romania 2University of Southern California, Ming Hsieh Department of Electrical Engineering, Los Angeles, CA 90089-2563, USA 3”Victor Babes¸” University of Medicine and Pharmacy Timis¸oara, Department of Biochemistry, Timis¸oara 300041, Romania 4University Politehnica of Timis¸oara, Department of Computer and Information Technology, Timis¸oara 300223, Romania 5University of Medicine and Pharmacy of Craiova, Faculty of Pharmacy, Craiova 200349, Romania 6Timis¸oara Institute of Complex Systems, Timis¸oara 300044, Romania *[email protected] ABSTRACT Despite recent advances in bioinformatics, systems biology, and machine learning, the accurate prediction of drug properties remains an open problem. Indeed, because the biological environment is a complex system, the traditional approach – based on knowledge about the chemical structures – cannot fully explain the nature of interactions between drugs and biological targets. Consequently, in this paper, we propose an unsupervised machine learning approach that uses the information we know about drug-target interactions to infer drug properties. -
Target Validation: a Door to Drug Discovery
Drug Discov Ther 2007;1(1):23-29. Review Target validation: A door to drug discovery Xiu-Ping Chen, Guan-Hua Du* Institute of Materia Medica, Chinese Academy of Medical Sciences and Peking Union Medical College, Beijing, China. ABSTRACT: From ancient times to today, a target is. The exact connotation of the term “drug drug discovery transitioned from serendipity to target” needs to be elucidated. Target validation is the rationality over its long history. Proper drug target first step in completely new drug discovery. Validation selection and validation are crucial to the discovery of new drug targets is the process of physiologically, of new drugs. This review discusses the definition pathologically, and pharmacologically evaluating a of drug targets and proposes several characteristics biomolecule and might be performed at the molecular, for drug targets. The limitations of the term ‘target’ cellular, or whole animal level. itself are summarized. The drug target validation Lectin-like oxidized low-density lipoprotein process is also discussed in detail and pitfalls during receptor-1 (LOX-1) was identified as a special receptor this process are outlined. Small active chemical for oxidized low-density lipoprotein (ox-LDL) in compounds obtained from the target validation endothelial cells (1). Accumulated studies revealed that process are useful tools in target validation and LOX-1 might play an important role in the pathogenesis target function research. The validation of lectin-like of atherosclerosis (2-7). Review of ten years of studies oxidized low-density lipoprotein receptor-1 (LOX-1) on LOX-1 suggested that LOX-1 might be a specific as a new potential anti-atherosclerotic drug target is new drug target, and validation results here revealed cited as an example in order to elucidate the target that LOX-1 is a new promising anti-atherosclerotic validation process.