Paleoredox and Pyritization of Soft-Bodied Fossils in the Ordovician Frankfort Shale of New York U´ Na C
Total Page:16
File Type:pdf, Size:1020Kb
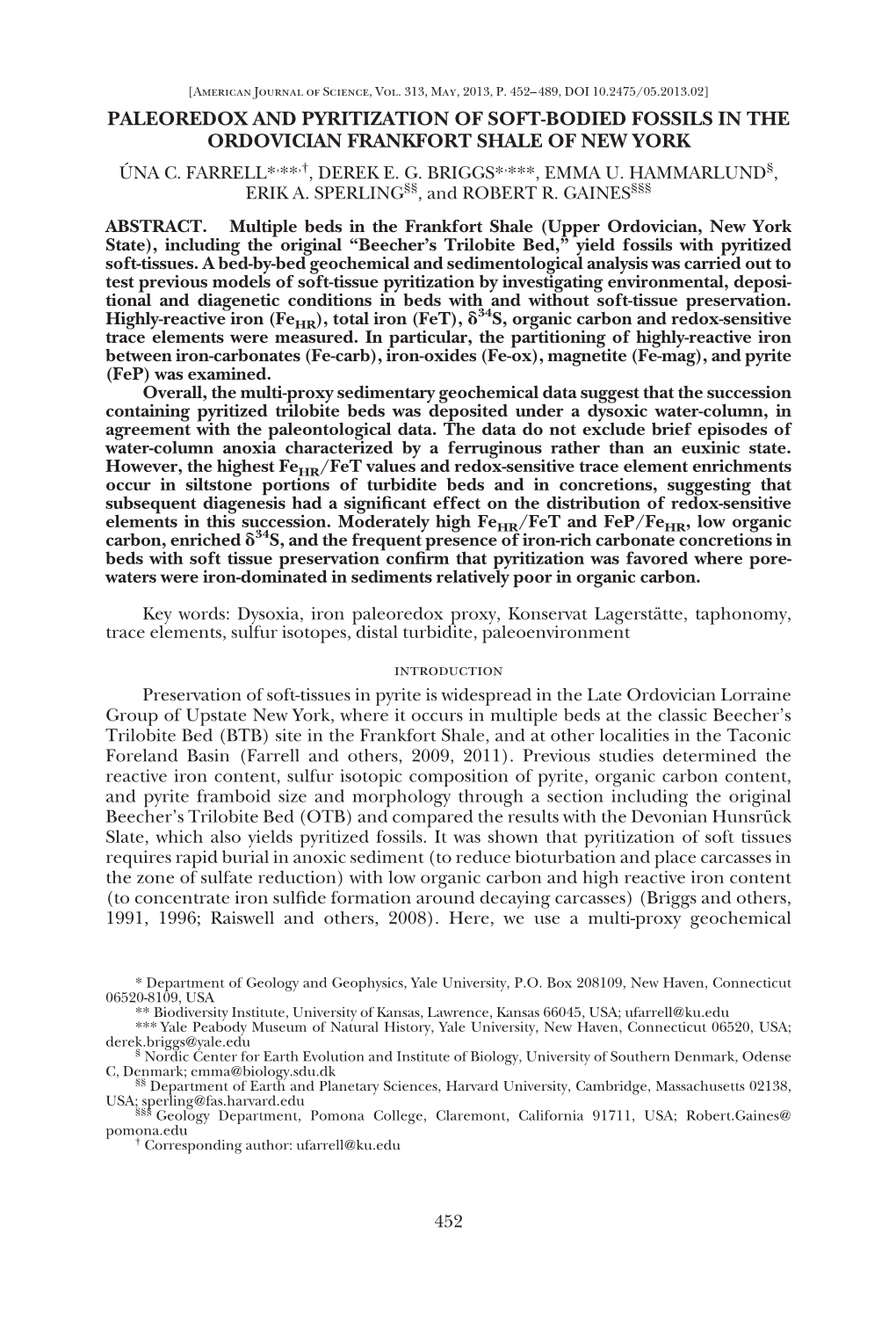
Load more
Recommended publications
-
The Classic Upper Ordovician Stratigraphy and Paleontology of the Eastern Cincinnati Arch
International Geoscience Programme Project 653 Third Annual Meeting - Athens, Ohio, USA Field Trip Guidebook THE CLASSIC UPPER ORDOVICIAN STRATIGRAPHY AND PALEONTOLOGY OF THE EASTERN CINCINNATI ARCH Carlton E. Brett – Kyle R. Hartshorn – Allison L. Young – Cameron E. Schwalbach – Alycia L. Stigall International Geoscience Programme (IGCP) Project 653 Third Annual Meeting - 2018 - Athens, Ohio, USA Field Trip Guidebook THE CLASSIC UPPER ORDOVICIAN STRATIGRAPHY AND PALEONTOLOGY OF THE EASTERN CINCINNATI ARCH Carlton E. Brett Department of Geology, University of Cincinnati, 2624 Clifton Avenue, Cincinnati, Ohio 45221, USA ([email protected]) Kyle R. Hartshorn Dry Dredgers, 6473 Jayfield Drive, Hamilton, Ohio 45011, USA ([email protected]) Allison L. Young Department of Geology, University of Cincinnati, 2624 Clifton Avenue, Cincinnati, Ohio 45221, USA ([email protected]) Cameron E. Schwalbach 1099 Clough Pike, Batavia, OH 45103, USA ([email protected]) Alycia L. Stigall Department of Geological Sciences and OHIO Center for Ecology and Evolutionary Studies, Ohio University, 316 Clippinger Lab, Athens, Ohio 45701, USA ([email protected]) ACKNOWLEDGMENTS We extend our thanks to the many colleagues and students who have aided us in our field work, discussions, and publications, including Chris Aucoin, Ben Dattilo, Brad Deline, Rebecca Freeman, Steve Holland, T.J. Malgieri, Pat McLaughlin, Charles Mitchell, Tim Paton, Alex Ries, Tom Schramm, and James Thomka. No less gratitude goes to the many local collectors, amateurs in name only: Jack Kallmeyer, Tom Bantel, Don Bissett, Dan Cooper, Stephen Felton, Ron Fine, Rich Fuchs, Bill Heimbrock, Jerry Rush, and dozens of other Dry Dredgers. We are also grateful to David Meyer and Arnie Miller for insightful discussions of the Cincinnatian, and to Richard A. -
The Larval Stages of Trilobites
THE LARVAL STAGES OF TRILOBITES. CHARLES E. BEECHER, New Haven, Conn. [From The American Geologist, Vol. XVI, September, 1895.] 166 The American Geologist. September, 1895 THE LARVAL STAGES OF TRILOBITES. By CHARLES E. BEECHEE, New Haven, Conn. (Plates VIII-X.) CONTENTS. PAGE I. Introduction 166 II. The protaspis 167 III. Review of larval stages of trilobites 170 IV. Analysis of variations in trilobite larvae 177 V. Antiquity of the trilobites 181 "VI. Restoration of the protaspis 182 "VII. The crustacean nauplius 186 VIII. Summary 190 IX. References 191 X Explanation of plates 193 I. INTRODUCTION. It is now generally known that the youngest stages of trilobites found as fossils are minute ovate or discoid bodies, not more than one millimetre in length, in which the head por tion greatly predominates. Altogether they present very little likeness to the adult form, to which, however, they are trace able through a longer or shorter series of modifications. Since Barrande2 first demonstrated the metamorphoses of trilobites, in 1849, similar observations have been made upon a number of different genera by Ford,22 Walcott,34':*>':t6 Mat thew,28- 27' 28 Salter,32 Callaway,11' and the writer.4.5-7 The general facts in the ontogeny have thus become well estab lished and the main features of the larval form are fairly well understood. Before the recognition of the progressive transformation undergone by trilobites in their development, it was the cus tom to apply a name to each variation in the number of tho racic segments and in other features of the test. -
Crossing Into Vancouver, BC, Is Easy. Don't Forget Your Passport
Crossing into Vancouver, BC, is easy. Don’t forget your passport. Tourism Vancouver / Capilano Suspension Bridge Park. JUNE | VOL. 24, 2014 6 NO. A PUBLICATION OF THE GEOLOGICAL SOCIETY OF AMERICA® JUNE 2014 | VOLUME 24, NUMBER 6 Featured Article GSA TODAY (ISSN 1052-5173 USPS 0456-530) prints news and information for more than 26,000 GSA member readers and subscribing libraries, with 11 monthly issues (April/ May is a combined issue). GSA TODAY is published by The SCIENCE: Geological Society of America® Inc. (GSA) with offices at 3300 Penrose Place, Boulder, Colorado, USA, and a mail- 4 An anthropogenic marker horizon in the future ing address of P.O. Box 9140, Boulder, CO 80301-9140, USA. rock record GSA provides this and other forums for the presentation of diverse opinions and positions by scientists worldwide, Patricia L. Corcoran, Charles J. Moore, and regardless of race, citizenship, gender, sexual orientation, Kelly Jazvac religion, or political viewpoint. Opinions presented in this publication do not reflect official positions of the Society. Cover: Plastiglomerate fragments interspersed with plastic debris, © 2014 The Geological Society of America Inc. All rights organic material, and sand on Kamilo Beach, Hawaii. Photo by K. Jazvac. reserved. Copyright not claimed on content prepared See related article, p. 4–8. wholly by U.S. government employees within the scope of their employment. Individual scientists are hereby granted permission, without fees or request to GSA, to use a single figure, table, and/or brief paragraph of text in subsequent work and to make/print unlimited copies of items in GSA TODAY for noncommercial use in classrooms to further education and science. -
Geologic Time, Concepts, and Principles
GEOLOGIC TIME, CONCEPTS, AND PRINCIPLES Sources: www.google.com en.wikipedia.org Thompson Higher Education 2007; Monroe, Wicander, and Hazlett, Physical orgs.usd.edu/esci/age/content/failed_scientific_clocks/ocean_salinity.html https://web.viu.ca/earle/geol305/Radiocarbon%20dating.pdf TCNJ PHY120 2013 GCHERMAN GEOLOGIC TIME, CONCEPTS, AND PRINCIPLES • Early estimates of the age of the Earth • James Hutton and the recognition of geologic time • Relative dating methods • Correlating rock units • Absolute dating methods • Development of the Geologic Time Scale • Geologic time and climate •Relative dating is accomplished by placing events in sequential order with the aid of the principles of historical geology . •Absolute dating provides chronometric dates expressed in years before present from using radioactive decay rates. TCNJ PHY120 2013 GCHERMAN TCNJ PHY120 2013 GCHERMAN Geologic time on Earth • A world-wide relative time scale of Earth's rock record was established by the work of many geologists, primarily during the 19 th century by applying the principles of historical geology and correlation to strata of all ages throughout the world. Covers 4.6 Ba to the present • Eon – billions to hundreds of millions • Era - hundreds to tens of millions • Period – tens of millions • Epoch – tens of millions to hundreds of thousands TCNJ PHY120 2013 GCHERMAN TCNJ PHY120 2013 GCHERMAN EARLY ESTIMATES OF EARTH’S AGE • Scientific attempts to estimate Earth's age were first made during the 18th and 19th centuries. These attempts all resulted in ages far younger than the actual age of Earth. 1778 ‘Iron balls’ Buffon 1710 – 1910 ‘salt clocks’ Georges-Louis Leclerc de Buffon • Biblical account (1600’S) 26 – 150 Ma for the oceans to become 74,832 years old and that as salty as they are from streams humans were relative newcomers. -
Pdf/17/3/932/5319294/932.Pdf 932 by Guest on 28 September 2021 Research Paper
Research Paper GEOSPHERE Late Pleistocene rates of rock uplift and faulting at the boundary between the southern Coast Ranges and the western Transverse GEOSPHERE, v. 17 no. 3 Ranges in California from reconstruction and luminescence dating https://doi.org/10.1130/GES02274.1 14 figures; 2 tables of the Orcutt Formation CORRESPONDENCE: [email protected] Ian S. McGregor and Nathan W. Onderdonk Department of Geological Sciences, California State University–Long Beach, 1250 Bellflower Boulevard, Long Beach, California 90804, USA CITATION: McGregor, I.S., and Onderdonk, N.W., 2021, Late Pleistocene rates of rock uplift and faulting at the boundary between the southern Coast Ranges ABSTRACT consistent with models that attribute shortening across the Santa Maria Basin and the western Transverse Ranges in California from to accommodation of clockwise rotation of the western Transverse Ranges and reconstruction and luminescence dating of the Orcutt Formation: Geosphere, v. 17, no. 3, p. 932–956, https:// The western Transverse Ranges and southern Coast Ranges of California suggest that rotation has continued into late Quaternary time. doi .org /10.1130 /GES02274.1. are lithologically similar but have very different styles and rates of Quaternary deformation. The western Transverse Ranges are deformed by west-trending Science Editor: Andrea Hampel folds and reverse faults with fast rates of Quaternary fault slip (1–11 mm/yr) ■ INTRODUCTION Associate Editor: Jeff Lee and uplift (1–7 mm/yr). The southern Coast Ranges, however, are primarily deformed by northwest-trending folds and right-lateral strike-slip faults with The Coast Ranges of California are deformed by northwest-striking faults Received 15 April 2020 Revision received 16 November 2020 much slower slip rates (3 mm/yr or less) and uplift rates (<1 mm/yr). -
Significant New Biostratigraphic Horizons in the Qusaiba Member of the Silurian Qalibah Formation of Central Saudi Arabia, and T
GeoArabia, Vol. 10, No. 1, 2005 Gulf PetroLink, Bahrain Significant new biostratigraphic horizons in the Qusaiba Member of the Silurian Qalibah Formation of central Saudi Arabia, and their sedimentologic expression in a sequence stratigraphic context Merrell A. Miller and John Melvin ABSTRACT Detailed analysis of over 1,000 subsurface Silurian palynology samples from 34 wells has allowed the development of a robust biostratigraphy based on acritarchs, chitinozoans and cryptospores for the Qusaiba Member of the Qalibah Formation, central Saudi Arabia. The new index fossils described herein augment the Arabian Plate Silurian chitinozoan zonation. The high-resolution biostratigraphic zonation consists of nine First Downhole Occurrences (FDOs) from the lower Telychian through Aeronian. In particular, three regionally recognizable palynologic horizons were identified within the lower part of the informally designated Mid-Qusaiba Sandstone (Angochitina hemeri Interval Zone), and above the FDO of Sphaerochitina solutidina. This high level of biostratigraphic resolution provides a framework for the integration of the sedimentology and calibration with global sea level curves, leading to a detailed understanding of the sequence stratigraphic evolution of this part of the Silurian in Saudi Arabia. Sedimentological core studies identify three Depositional Facies Associations (DFAs) within the Mid-Qusaiba Sandstone interval, including: (1) shelfal deposits (DFA-I) characterized by interbedded hummocky cross-stratified sandstones, graded siltstones and bioturbated mudstones; (2) turbiditic deposits (DFA-II); and (3) an association of heavily contorted and re- sedimented sandstones, siltstones and mudstones (DFA-III) that is considered representative of oversteepened slopes upon the Qusaiba shelf. Integration of the newly recognized palynostratigraphic horizons and the sedimentological data facilitates an understanding of the sequence stratigraphic evolution of the Mid-Qusaiba Sandstone interval and its immediate precursors. -
Stratigraphy and Sedimentology of the Upper Cretaceous to Paleogene Kilwa Group, Southern Coastal Tanzania
Available online at www.sciencedirect.com SCIENCELNCE^I (ri\ DIRECT* journal of African Earth Sciences ELSEVIER Journal of African Earth Sciences 45 (2006) 431-466 www.elsevier.com/locate/jafrearsci Stratigraphy and sedimentology of the Upper Cretaceous to Paleogene Kilwa Group, southern coastal Tanzania Christopher J. Nicholas a*, Paul N. Pearson b, Paul R. Bown c, Tom Dunkley Jones Brian T. Huber d, Amina Karega e, Jackie A. Leesc, Ian K. McMillan b, Aoife O'Halloran a, Joyce M. Singano e, Bridget S. Wade f a Department of Geology, Trinity College, University of Dublin, Dublin 2, Ireland School of Earth, Ocean and Planetary Sciences, Cardiff University, Main Building, Park Place, Cardiff CF10 3 YE, United Kingdom c Department of Earth Sciences, University College London, Gower Street, London WC1E 6BT, United Kingdom Department of Paleobiology, MRC NHB-121, P.O. Box 37012, Smithsonian National Museum of Natural History, 10th and Constitution Avenue, Washington, DC 20012-7012, USA e Tanzania Petroleum Development Corporation, P.O. Box 2774, Dar-es-Salaam, Tanzania Department of Geological Sciences, Rutgers, The State University of New Jersey, Wright Laboratory, Busch Campus, Received 10 October 2005; received in revised form 13 March 2006; accepted 7 April 2006 Available online 13 June 2006 Abstract The geology of southern coastal Tanzania has remained poorly understood since the first comments on its stratigraphy were made over 100 years ago. However, new field surveys combined with shallow drilling along the coast between Kilwa and Lindi are beginning to resolve the depositional history and structural evolution of this region over the past 85 Ma. -
Resolving Details of the Nonbiomineralized Anatomy of Trilobites Using Computed
Resolving Details of the Nonbiomineralized Anatomy of Trilobites Using Computed Tomographic Imaging Techniques Thesis Presented in Partial Fulfillment of the Requirements for the Master of Science in the Graduate School of The Ohio State University By Jennifer Anita Peteya, B.S. Graduate Program in Earth Sciences The Ohio State University 2013 Thesis Committee: Loren E. Babcock, Advisor William I. Ausich Stig M. Bergström Copyright by Jennifer Anita Peteya 2013 Abstract Remains of two trilobite species, Elrathia kingii from the Wheeler Formation (Cambrian Series 3), Utah, and Cornuproetus cornutus from the Hamar Laghdad Formation (Middle Devonian), Alnif, Morocco, were studied using computed tomographic (CT) and microtomographic (micro-CT) imaging techniques for evidence of nonbiomineralized alimentary structures. Specimens of E. kingii showing simple digestive tracts are complete dorsal exoskeletons preserved with cone-in-cone concretions on the ventral side. Inferred stomach and intestinal structures are preserved in framboidal pyrite, likely resulting from replication by a microbial biofilm. C. cornutus is preserved in non- concretionary limestone with calcite spar lining the stomach ventral to the glabella. Neither species shows sediment or macerated sclerites of any kind in the gut, which tends to rule out the possibilities that they were sediment deposit-feeders or sclerite-ingesting durophagous carnivores. Instead, the presence of early diagenetic minerals in the guts of E. kingii and C. cornutus favors an interpretation of a carnivorous feeding strategy involving separation of skeletal parts of prey prior to ingestion. ii Dedication This manuscript is dedicated to my parents for encouraging me to go into the field of paleontology and to Lee Gray for inspiring me to continue. -
The Pennine Lower and Middle Coal Measures Formations of the Barnsley District
The Pennine Lower and Middle Coal Measures formations of the Barnsley district Geology & Landscape Southern Britain Programme Internal Report IR/06/135 BRITISH GEOLOGICAL SURVEY GEOLOGY & LANDSCAPE SOUTHERN BRITAIN PROGRAMME INTERNAL REPORT IR/06/135 The Pennine Lower and Middle Coal Measures formations of the Barnsley district The National Grid and other R D Lake Ordnance Survey data are used with the permission of the Controller of Her Majesty’s Stationery Office. Editor Licence No: 100017897/2005. E Hough Keywords Pennine Lower Coal Measures Formation; Pennine Middle Coal Measures Formation; Barnsley; Pennines. Bibliographical reference R D LAKE & E HOUGH (EDITOR).. 2006. The Pennine Lower and Middle Coal Measures formations of the Barnsley district. British Geological Survey Internal Report,IR/06/135. 47pp. Copyright in materials derived from the British Geological Survey’s work is owned by the Natural Environment Research Council (NERC) and/or the authority that commissioned the work. You may not copy or adapt this publication without first obtaining permission. Contact the BGS Intellectual Property Rights Section, British Geological Survey, Keyworth, e-mail [email protected]. You may quote extracts of a reasonable length without prior permission, provided a full acknowledgement is given of the source of the extract. Maps and diagrams in this book use topography based on Ordnance Survey mapping. © NERC 2006. All rights reserved Keyworth, Nottingham British Geological Survey 2006 BRITISH GEOLOGICAL SURVEY The full range of Survey publications is available from the BGS British Geological Survey offices Sales Desks at Nottingham, Edinburgh and London; see contact details below or shop online at www.geologyshop.com Keyworth, Nottingham NG12 5GG The London Information Office also maintains a reference 0115-936 3241 Fax 0115-936 3488 collection of BGS publications including maps for consultation. -
Yaxcopoil-1 and the Chicxulub Impact
Int J Earth Sci (Geol Rundsch) (2004) 93: 1042–1065 First published in: DOI 10.1007/s00531-004-0431-6 ORIGINAL PAPER Wolfgang Stinnesbeck Æ Gerta Keller Æ Thierry Adatte Markus Harting Æ Doris Stu¨ben Æ Georg Istrate Utz Kramar Yaxcopoil-1 and the Chicxulub impact Received: 28 August 2003 / Accepted: 11 July 2004 / Published online: 29 October 2004 Ó Springer-Verlag 2004 Abstract CSDP core Yaxcopoil-1 was drilled to a depth Cenomanian organic-rich marly limestone. There is no of 1,511 m within the Chicxulub crater. An organic-rich evidence that these sediments represent crater infill due marly limestone near the base of the hole (1,495 to to megablocks sliding into the crater, such as major 1,452 m) was deposited in an open marine shelf envi- disruption of sediments, chaotic changes in lithology, ronment during the latest Cenomanian (uppermost overturned or deep dipping megablocks, major Rotalipora cushmani zone). The overlying sequence of mechanical fragmentation, shock or thermal alteration, limestones, dolomites and anhydrites (1,495 to 894 m) or ductile deformation. Breccia units that are interca- indicates deposition in various carbonate platform lated in the carbonate platform sequence are intrafor- environments (e.g., sabkhas, lagoons). A 100-m-thick mational in origin (e.g., dissolution of evaporites) and suevite breccia (894–794 m) identifies the Chicxulub dykes are rare. Major disturbances of strata by the im- impact event. Above the suevite breccia is a dolomitic pact therefore appear to have been confined to within limestone with planktic foraminiferal assemblages less than 60 km from the proposed impact center. -
New Developments Regarding the KT Event and Other Catastrophes in Earth History
NASA-CR-195169 ///_/- _/_ " C-'_/#_'/ NEW OEVELOPMENTS N94-28294 EVENT AND OTHER --THRU-- EARTH HISTORY N94-28314 150 p Unclas G3/46 0208810 ...o_ep]F1)_'F" PAPERS PRESENTED TO New Developments Regarding the KT Event and Other Catastrophes in Earth History FEBRUARY 9-12, 1994 • HOUSTON, TEXAS PAPERS PRESENTED TO NEW DEVELOPMENTS REGARDING THE KT EVENT AND OTHER CATASTROPHES IN EARTH HISTORY February 9-12, 1994 Houston, Texas Sponsored by Lunar and Planetary Institute University of Houston-Clear Lake L P I _/ear L__'_ LPI Contribution No. 825 Compiled in 1994 by LUNAR AND PLANETARY INSTITUTE The Institute is operated by the University Space Research Association under Contract No. NASW-4574 with the National Aeronautics and Space Administration. Material in this volume may be copied without restraint for library, abstract service, education, or personal research purposes; however, republication of any paper or portion thereof requires the written permission of the authors as well as the appropriate acknowledgment of this publication. This report may be cited as Author A. B. (1994) Title of abstract. In New Developments Regarding the KT Event and Other Catastrophes in Earth History. LPI Contribution No. 825, Lunar and Planetary Institute, Houston. 138 pp. This report is distributed by ORDER DEPARTMENT Lunar and Planetary Institute 3600 Bay Area Boulevard Houston TX 77058-1113 Mail order requestors will be invoiced for the cost of shipping and handling. LPI Contribution No. 825 iii Preface This volume contains papers that have been accepted for presentation at the conference on New Developments Regarding the KT Event and Other Catastrophes in Earth History, February 9-12, 1994, in Houston, Texas. -
The Influence of Sea Level and Tectonics on Late Pleistocene
ARTICLE IN PRESS MARGO-04409; No of Pages 21 Marine Geology xxx (2009) xxx–xxx Contents lists available at ScienceDirect Marine Geology journal homepage: www.elsevier.com/locate/margeo The influence of sea level and tectonics on Late Pleistocene through Holocene sediment storage along the high-sediment supply Waipaoa continental shelf Thomas P. Gerber a,⁎, Lincoln F. Pratson a, Steve Kuehl b, J.P. Walsh c, Clark Alexander d, Alan Palmer e a Nicholas School of the Environment and Earth Sciences, Old Chemistry Box 90227, Duke University, Durham, NC 27708, USA b Virginia Institute of Marine Sciences, Rt.1208, Greate Road, P.O. Box 1346, Gloucester Point, VA 23062, USA c Department of Geological Sciences, East Carolina University, Room 101 Graham Building, Greenville, NC 27858, USA d Skidaway Institute of Oceanography, 10 Ocean Science Circle, Savannah, GA 31411, USA e Soil and Earth Sciences, Institute of Natural Resources, Private Bag 11 222, Palmerston North, New Zealand article info abstract Available online xxxx We present geophysical and core evidence showing how subsidence caused by forearc shortening has accommodated Late Pleistocene and Holocene sediments supplied to the tectonically active Waipaoa shelf Keywords: (NZ), limiting off-shelf export during the early sea level highstand. The last glacioeustatic fall and subsequent Waipaoa shelf rise exposed and then flooded a shelf segmented into subbasins separated by zones of uplift, leaving key stratigraphy stratigraphic markers of shoreline regression and transgression that vary strongly in character across the transgression shelf. Highstand sediment isopachs tied to piston cores dated using tephra correlation and a radiocarbon age highstand model provide a sediment budget at ∼2000yr intervals from the mid-Holocene (∼5500 cal.yrBP) to present.