Strategies to Combat Inflammation in Pancreas and Islet Transplantation
Total Page:16
File Type:pdf, Size:1020Kb
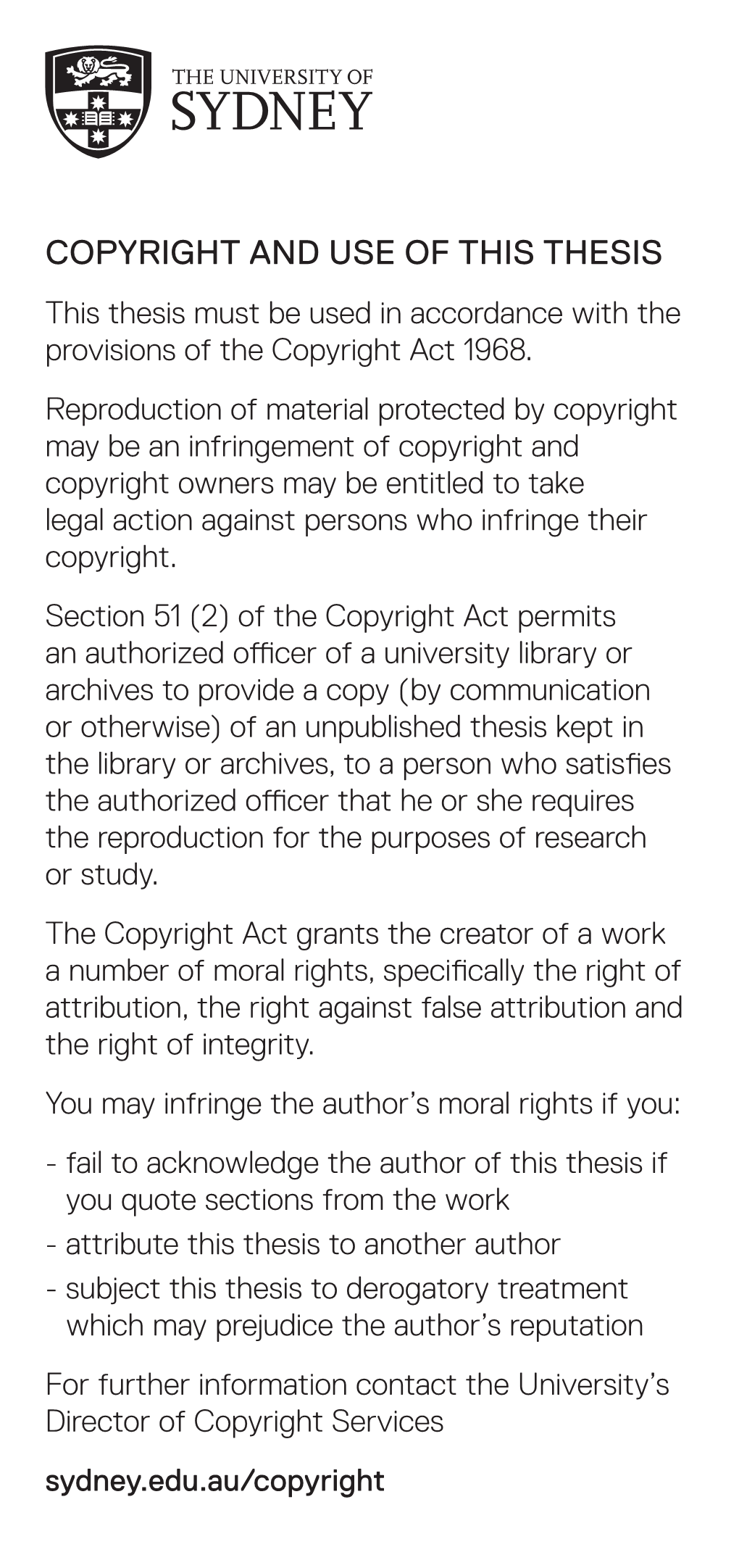
Load more
Recommended publications
-
What Every Physician Should Know About Doping and Doping Control O Que Todo Médico Deveria Conhecer Sobre Doping E Controle Antidoping
WHATCOMMUNICATION EVERY PHYSICIAN SHOULD KNOW ABOUT DOPING AND DOPING CONTROL What every physician should know about doping and doping control O QUE TODO MÉDICO DEVERIA CONHECER SOBRE DOPING E CONTROLE ANTIDOPING JOSÉ KAWAZOE LAZZOLI1 1Adjunct Professor, Instituto Biomédico, Universidade Federal Fluminense. Specialist in Sports Medicine from Sociedade Brasileira de Medicina do Exercício e do Esporte (SBMEE) and Cardiology from Sociedade Brasileira de Cardiologia (SBC). Former President of the Brazilian Society of Sports Medicine (2009/2011). Secretary General of the Panamerican Confederation of Sports Medicine (Copamede). Treasurer of the International Federation of Sports Medicine (FIMS), Rio de Janeiro, RJ, Brazil http://dx.doi.org/10.1590/1806-9282.62.02.101 Probably the medical professional who is closer to the teria are present in a substance or a method, the theoret- daily life of an athlete, who participates regularly in com- ical possibility of listing occurs. petitions, whether or not high-performance, is the expert Examples of artificial performance enhancement in- in exercise and sports medicine. However, occasionally clude anabolic steroids, which also cause serious risks to the athlete may need to resort to other medical profes- the health of the athlete. This group of substances in- sionals, for various reasons, such as a general practitio- cludes two of the three basic principles of the concept of ner, a dermatologist, a cardiologist, an orthopedist or a doping, and therefore makes the list. gastroenterologist. Therefore, every physician is subject The concept of “violating the spirit of sport” can give to come across a patient who is an intermediate level or rise to subjective interpretations, but these values are ex- high-performance athlete, and will need to go through plained in WADA World Anti-Doping Code as follows: eth- doping controls throughout his sporting life. -
Freediving Catalog 2014
Freediving 2014 760B2EG 760B3EG 760B4EG 760B5EG E-GLASS DESCRIPTION Our most popular and all around bifins. The lenght of the blade is compatible with dynamic and constant weight apnea. Composite blades to stock up on maximum energy throughout the bending. TECHNOLOGY Technology : prepreg curing process Fabric : E-glass Resin : epoxy Performance : 30 to 40% more responsive than plastic SPECIFICATIONS Height of blade : 760 mm Width of blade : 210 mm Flat blade for made-to-measure footpocket (free heel) Blade with an angle of 15° to fit in full footpocket 4 kinds of varying hardness that are most likely to suit your style and body type : 760B2EG : soft, dynamic apnea 760B3EG : medium, dynamic and constant weight apnea 760B4EG : hard, constant weight 760B5EG : very hard, constant weight for big guys + 90kg Weight with made-to-measure footpocket = 1,5kg Weight with full footpocket = 1,9kg T profiles along the edges to ensure a good drive and a channeling of the water FOOTPOCKETS 2 kinds of footpockets : - Made-to-measure footpocket : Size 35 to 50 (3 to 15), free heel for better stroke - Tuned full footpocket : cut out footpocket to get a lighter and more responsive swimfin. Beuchat Mundial : 41-42, 43-44, 45-46, 47-48 Imersion : 38-40, 40-42, 42-44, 44-46 Omer : 36-38, 38-40, 40-42, 42-44, 44-46, 46-48, 48-50 760B2SG 760B3SG 760B4SG 760B5SG S-GLASS DESCRIPTION Our most popular and all around bifins. The lenght of the blade is compatible with dynamic and constant weight apnea. Composite blades to stock up on maximum energy throughout the bending. -
International Handbook on Tourism and Peace
CORDULA WOHLMUTHER WERNER WINTERSTEINER (Eds.) International Handbook on Tourism and Peace Centre for Peace Research and Peace Education of the Klagenfurt University/ Austria in cooperation with the World Tourism Organization ( UN WTO ) DRAVA Disclaimer: e opinions contained in this publication are exclusively those of the authors and do not necessarily reect the point of view of the editors or the UNWTO. e designations employed and the presentation of material in this publication do not represent any opinions whatsoever on the part of the Centre for Peace Research and Peace Education of Klagenfurt Uni- versity or the Secretariat of the World Tourism Organization concerning the legal status of any country, territory, city or area, or of its au thorities or concerning the delimitation of its frontiers or boundaries. is book is the Yearbook Culture of Peace , edited by the Centre of Peace Research and Peace Education of the Klagenfurt University/Austria · Klagenfurt/Celovec, Austria www.drava.at © Copyright by Drava Verlag/Založba Drava Cover design by Walter Oberhauser, cover art by Sim Jun Han Interior design and printing by Drava Print GmbH ---- CORDULA WOHLMUTHER WERNER WINTERSTEINER Eds. International Handbook on Tourism and Peace Centre for Peace Research and Peace Education of the Klagenfurt University/ Austria in cooperation with World Tourism Organization ( UN WTO ) DRAVA Centre for Peace Research and Peace Education Printed with the support of: Forschungsrat of Klagenfurt University with sponsoring provided by the Carinthian Federal State Government Swedish Tourism Innovation Centre Austrian Federal Ministry for European and International Aairs Austrian Federal Ministry for Education, Arts and Culture TPA-Horwath Group, Tax Advisory and Auditing Services Kärntner Universitätsbund is book is published within the framework of the Project “Tourism and Peace”, an Initiative of the World Tourism Organization (UNWTO) and the Klagenfurt University, Austria and its Centre for Peace Research and Peace Education. -
The Minnesota Electrical Association, Inc. the Voice of Electrical Contractors
The Minnesota Electrical Association, Inc. The Voice of Electrical Contractors www.electricalassociation.com Electrical Toolbox Talks The MEA Safety Committee is pleased to present you with your set of Electrical Toolbox Talks. This collection of safety topics is a collaborative effort betweenMEA’s Safety Committee, Federated Insurance Associated Risk Management Services Team, and Minnesota OSHA Consultation. This is a series of short, concise, industry specific topics. We are including a sign-in form for OSHA documentation; please make copies of this sheet as needed. These talks may be used as topics for your short safety meetings, as a salary stuffer for your employees, or as a handout at your safety meetings. Your new Electrical Toolbox Talks topics are enclosed: • Aerial Lifts • Emergency Preparedness • Ladder Safety • Arc Blasts • Excavations • Liquefied Petroleum Gas • Asbestos • Extension Cords • Lockout/Tagout • Back Safety • Eye and Face Safety • Power Tools & Equipment Safety • Bloodborne Pathogen Safety • Fall Protection • PPE Assessment • Boom Trucks • Fatigue • PPE for Respirators • Carbon Monoxide • Fire Extinguisher Safety • Safe Lifting & Material Handling • Cold Stress • Foot Protection • Safety Data Sheets • Competent vs. Qualified Person • Fork Lift Drivers • Scaffolding Safety • Compressed Gas Safety • Guardrail Requirements • Security Safety • Confined Space Safety • Hand Safety • Temporary Heating • Construction Sites • Head Protection • Temporary Lighting • Controlling Electrical Hazards • Hearing Safety • Temporary Wiring Panels • Core Drilling • Heat Stress • Trips, Slips, and Falls • Crystalline Silica • High-Visibility Vests • Winter Driving • Driving Safety • Injuries & Emergency Situations Acknowledgements: MEA Safety Committee Members, including our MN OSHA Consultation team members Andy Smoka and Tim Brown, and our Federated Insurance team members Jeff Stevenson and Pat Swetala. -
Animal Genetic Resources Information Bulletin
The designations employed and the presentation of material in this publication do not imply the expression of any opinion whatsoever on the part of the Food and Agriculture Organization of the United Nations concerning the legal status of any country, territory, city or area or of its authorities, or concerning the delimitation of its frontiers or boundaries. Les appellations employées dans cette publication et la présentation des données qui y figurent n’impliquent de la part de l’Organisation des Nations Unies pour l’alimentation et l’agriculture aucune prise de position quant au statut juridique des pays, territoires, villes ou zones, ou de leurs autorités, ni quant au tracé de leurs frontières ou limites. Las denominaciones empleadas en esta publicación y la forma en que aparecen presentados los datos que contiene no implican de parte de la Organización de las Naciones Unidas para la Agricultura y la Alimentación juicio alguno sobre la condición jurídica de países, territorios, ciudades o zonas, o de sus autoridades, ni respecto de la delimitación de sus fronteras o límites. All rights reserved. No part of this publication may be reproduced, stored in a retrieval system, or transmitted in any form or by any means, electronic, mechanical, photocopying or otherwise, without the prior permission of the copyright owner. Applications for such permission, with a statement of the purpose and the extent of the reproduction, should be addressed to the Director, Information Division, Food and Agriculture Organization of the United Nations, Viale delle Terme di Caracalla, 00100 Rome, Italy. Tous droits réservés. Aucune partie de cette publication ne peut être reproduite, mise en mémoire dans un système de recherche documentaire ni transmise sous quelque forme ou par quelque procédé que ce soit: électronique, mécanique, par photocopie ou autre, sans autorisation préalable du détenteur des droits d’auteur. -
COOPERATIVE INSTITUTE for RESEARCH in ENVIRONMENTAL SCIENCES University of Colorado at Boulder UCB 216 Boulder, CO 80309-0216
CELEB ra TING 0 OF ENVI R ONMENT A L RESE arc H years 4 COOPERATIVE INSTITUTE FOR RESEARCH ANNU A L REPO R T IN ENVIRONMENTAL SCIENCES University of Colorado at Boulder i 00 2 8 COOPERATIVE INSTITUTE FOR RESEARCH IN ENVIRONMENTAL SCIENCES University of Colorado at Boulder UCB 216 Boulder, CO 80309-0216 Phone: 303-492-1143 Fax: 303-492-1149 email: [email protected] http://cires.colorado.edu ANNU A L REPO R T ST A FF Suzanne van Drunick, Coordinator Jennifer Gunther, Designer Katy Human, Editor COVE R PHOTO Pat and Rosemarie Keough part of traveling photographic exhibit Antarctica—Passion and Obsession Sponsored by CIRES in celebration of 40th Anniversary ii From the Director 2 Executive Summary and Research Highlights 4 The Institute Year in Review 12 Contributions to NOAA’s Strategic Vision 13 Administration and Funding 16 Creating a Dynamic Research Environment 21 CIRES People and Projects 26 Faculty Fellows Research 27 Scientific Centers 58 Education and Outreach 68 Visiting Fellows 70 Innovative Research Projects 73 Graduate Student Research Fellowships 83 Diversity and Undergraduate Research Programs 85 Theme Reports 86 Measures of Achievement: Calendar Year 2007 146 Publications by the Numbers 147 Refereed publications 148 Non-refereed Publications 172 Refereed Journals in which CIRES Scientists Published 179 Honors and Awards 181 Service 185 Appendices 188 Governance and Management 189 Personnel Demographics 193 Acronyms and Abbreviations 194 CIRES Annual Report 2008 1 From the Director 2 CIRES Annual Report 2008 am very proud to present the new CIRES annual report for fiscal year 2008. It has been another exciting year with numerous accomplishments, Iawards, and continued growth in our research staff and budget. -
Federico Mana -100
SHARM october . 2009 . COMPLEMENTARY COPY VOLUME 016 TIME EXCELLENCY DEED Only One Apnea Centre, a record factory in Sharm: Federico Mana -100 - You feel very small, almost insig- the calm waters of Sodfa, straight nificant being down there…- said in front of the Sharm Club Hotel. Federico Mana being back from 100 Ten humans only in the world can metres depth holding his breath. say: I have been that deep without Cristal clear waters, excellent weath- the help of a sled to descend, only er conditions, and a mild in water relying on my fins and lungs - and temperature all year round, are an now Federico is one of them. In a BLUE TIME outstanding setting for outstanding three minutes dive Federico swam performances in free-diving training. down to the depth to 40 metres, For these reasons Umberto Pelizzari, where his body become less buoy- the Italian free-diving living legend, ant, and sunk to the abyssal depth in 2003 decided to start in Sharm el of one hundred metres. He swam Sheikh the Red Sea branch of his up again holding the -100 tag in his Apnea Academy, the prestigious free hand, a timeless swim toward the diving school and instructor develop- surface, and the air. From the float- ment agency. ing platform two AIDA judges eval- - The Red Sea has fantastic reefs, is uated Federico’s performance and, within easy reach from Europe, it of- after the canonical thirty seconds, fers the possibility to find current-free issued their verdict: Federico Mana spots, a very important issue when is the new Italian Record holder. -
On Health Risks of Ambient PM in the Netherlands
Section 0 0 - 1 On health risks of ambient PM in the Netherlands Full Report Netherlands Aerosol Programme October 2002 RIVM report 650010 032 Editors: Eltjo Buringh and Antoon Opperhuizen Authors: Aben, J.1;Ameling, C.B.1; Beck, J.1; Boere, A.J.F.; Breugel, P.B. van1; Brink, H.M. ten3; Brink, R.M.M. van den1; Buijsman, E.1; Brunekreef, B.4; Buringh, E.1; Cassee, F.R.1; Dekkers, A.L.M.1; Dolmans, J.1; Eerens, H.C.1; Fischer, P.H.1; Harmelen, A.K. van2; Keuken, M.P.2; Kooter, I.M.1; Loon, M. van2; Loveren, H. van1; Marra, M.1; Matthijsen, J.1; Noordijk, H.1; Opperhuizen, A.1; Schaap, M.2,3; Schlesinger, R.B.5; Slanina, J.3; Smeets, P.1; Smeets, W.L.M.1; Spoelstra, H.2; Steerenberg, P.A.1; Visschedijk, A.J.H.2; Visser, H.1; Vries, W.J. de1; Weijers, E.3; Winter, R. de1; 1) Rijksinstituut voor Volksgezondheid en Milieu, Bilthoven 2) Nederlandse Organisatie voor Toegepast Natuurwetenschappelijk Onderzoek, Apeldoorn 3) Energieonderzoek Centrum Nederland, Petten 4) Institute of Risk Assessment Studies, University of Utrecht 5) Department of Biological Sciences, Pace University, Pleasantville, NY, USA On health risks of ambient PM in the Netherlands Full Report October 2002 Section 0 0 - 2 Abstract Particulate Matter (PM) in the ambient air can lead to health effects and even to premature mortality. This result has been found in a score of epidemiological studies, but their cause is not yet clear. It is certain, however, that these effects are so serious and so extensive that further action is warranted. -
Monofin 2015
MONOFIn 2015 THE SWIMFINS COMPANY ME1EG / ME2EG / ME3EG DESCRIPTION A very good monofin introduction for childs. A small blade to avoid strain. Made-to-measure footpocket starting at size 3 TECHNOLOGY Technology : prepreg curing process Fabric : E-glass Resin : epoxy Performance : 30 to 40% more responsive than plastic SPECIFICATIONS Height of blade : 600 mm Width of blade : 600 mm Surface area of the pair of blades : 2700 cm2 Flat blade for made-to-measure footpocket (free heel) Blade with an angle of 15° to fit in full footpocket 3 kinds of varying hardness that are most likely to suit your style and body type : ME1EG : soft ME2EG : medium ME3EG : hard Weight with made-to-measure footpocket = 1,5kg Weight with full footpocket = 1,9kg U-shaped sections along the edge to ensure a good protection of the blade and the swimmer FOOTPOCKETS 2 kinds of footpockets : - Made-to-measure footpocket : Size 35 to 50 (3 to 15), free heel for better stroke optional extra : velcro strap for instep fine tuning - Tuned full footpocket : cut out footpocket to get a lighter and more responsive swimfin. Imersion : 38-40, 40-42, 42-44, 44-46 Pathos : 36-38, 38-40, 40-42, 42-44, 44-46, 46-48, 48-50 ME1SG / ME2SG / ME3SG DESCRIPTION A very good monofin introduction for childs. A small blade to avoid strain. Made-to-measure footpocket starting at size 3 Better strength and improved resistance to impact and crack propagation thanks to S Glass. TECHNOLOGY Technology : prepreg Fabric : S-glass fibre Resin : epoxy Performance : 4 to 5% more responsive than E-glass -
Advocate DOSH
January-March 2014 Arizona Division of Occupational Safety & Health Volume 1 Upcoming Events Water/Wastewater Worker Safety Seminar and Nogales February 5th - Nogales Water Treatment Plant ADOSH Regional Safety Summit Mohave Community DOSH College - Kingman Campus February 19-20 Advocate Looking Ahead: Auto Shop Safety April 9th in Bullhead City Inside this issue: Winter Conditions Ahead...Proceed with Care! Winter Conditions Ahead - 1 Proceed with Care! Not all of Arizona is bathed in sun- • Train the workforce about cold- shine all winter long; indeed, some induced illnesses and injuries. Kingman Safety Summit 2 areas are blanketed with snow from OSHA has prepared an employee February 19-20, 2014 time to time and blasted with icy training aid to help you present winds that curl your toes and bite this essential information. Regular Features your nose! 3 www.osha.gov/Publications/osha A Thousand Words Consider our own Winter Wonder- 3156.pdf (see picture at right) In-Box lands: Flagstaff, White Mountains, Prescott, Williams, Mt. Lemon and • Select proper clothing for cold, Arizona’s Medical Marijuana 4 wet, and windy conditions. Layer Act in the Workplace even the Valley of the Sun was by Gina Kesler Pres. D&A Experts dusted with snow last February clothing to adjust to changing envi- ronmental temperatures. Wear a hat and gloves, in addition to un- Trainer’s Corner - Power 5 Points... What’s the Point? derwear that will keep water away from the skin (polypropylene). The Employer’s Mandate to Protect Temporary Workers • Take frequent short breaks in warm dry shelters to allow the Training Calendar 6- body to warm up. -
Should Antiarrhythmic Treatment Be Considered Doping in a Shooting Athlete? Renata Rodrigues Teixeira De Castro1,2 ,*
CLINICAL ARRHYTHMIA https://doi.org/10.24207/jca.v34i1.3411 Case Report Should Antiarrhythmic Treatment Be Considered Doping in a Shooting Athlete? Renata Rodrigues Teixeira de Castro1,2 ,* ORCID ID Castro RRT https://orcid.org/0000-0001-5560-693X ABSTRACT Beta-blockers are prohibited for athletes competing in particular sports. This article presents a case of sick sinus syndrome in a master shooting athlete and discuss the potential shooting accuracy enhancement obtained by the treatment beyond beta-blockade. KEYWORDS: Doping; Beta-blocker; Shooting; Pacemaker; Performance. INTRODUCTION Doping refers to the use or intended use of prohibited substances and/or methods according to the Prohibited List of the International Standard of the World Anti-Doping Agency, which is reviewed and updated at least once a year1. Prohibited drugs and methods are not the same for all sports. Whereas some athletes would benefit from increased power or speed, others may achieve better results through better steadiness and accuracy. This is the case of archery, shooting and other particular sports (Table 1), where the use of beta-blockers reduces heart rate and muscle tremor, consequently improving performance2. Although various drugs and even pacemakers can keep heart rate in a slow pace, only beta-blockers are considered doping. One usually imagines that athletes are young and healthy, but this is not always true. While the average age of Olympic athletes in London Olympic Games (2012) was 26, there were 187 athletes over 40 years old in London Olympic Games. Most over-50s were competing in equestrian and shooting, and all of them were subject to doping control. -
Management Support for Safety: Disrupting the Paradigm Scotty Dunlap Eastern Kentucky University
Eastern Kentucky University Encompass EKU Faculty and Staff choS larship Spring 2019 Management support for safety: Disrupting the paradigm Scotty Dunlap Eastern Kentucky University Follow this and additional works at: https://encompass.eku.edu/fs_research Recommended Citation Dunlap, S. (2019). Management support for safety: Disrupting the paradigm. Safety Decisions. (Spring/Summer, 22-24) This Article is brought to you for free and open access by Encompass. It has been accepted for inclusion in EKU Faculty and Staff choS larship by an authorized administrator of Encompass. For more information, please contact [email protected]. BEYOND COMPLIANCE: TAKING CARE OF THE SAFETY PROFESSIONAL p34 Spring/Summer 2019 SafetyDecisionsMagazine.com Management OSHA Support for Safety: Explains Disrupting the Hybrid Paradigm p22 SDSs p43 Unaffected by Government Shutdown, OSHA Increases Penalties p48 We Can’t Fix Mental Health Lockout/ Tagout: Are with Duct Tape: There Changes on A New Frontier the Horizon? p30 in Safety p12 Spring/Summer 2019 Contents VOLUME V n NUMBER 2 DEPARTMENTS 40 Employers See the Effect of Rises in Marijuana and Opioid Use The use and misuse of both illicit and Keeping Up prescription drugs are affecting a growing 6 Beryllium Enforcement … Avetta and number of employers. BROWZ Merge … NTSB’s ‘Most Wanted’ By Guy Burdick … Whistleblower ADR Policy … Carbon Monoxide … Telgian’s CFATS Software … Nurses and PPE … NIOSH Resources … 43 OSHA Explains Hybrid SDSs Wal-Mart Citation Vacated … Still confused about some of the finer Defense Contractor Safety Violations … points of the HazCom and GHS standards? You’re not alone, and OSHA recently provided some guidance. Strategy By William C.