Silicene-Like Domains on Irsi3 Crystallites
Total Page:16
File Type:pdf, Size:1020Kb
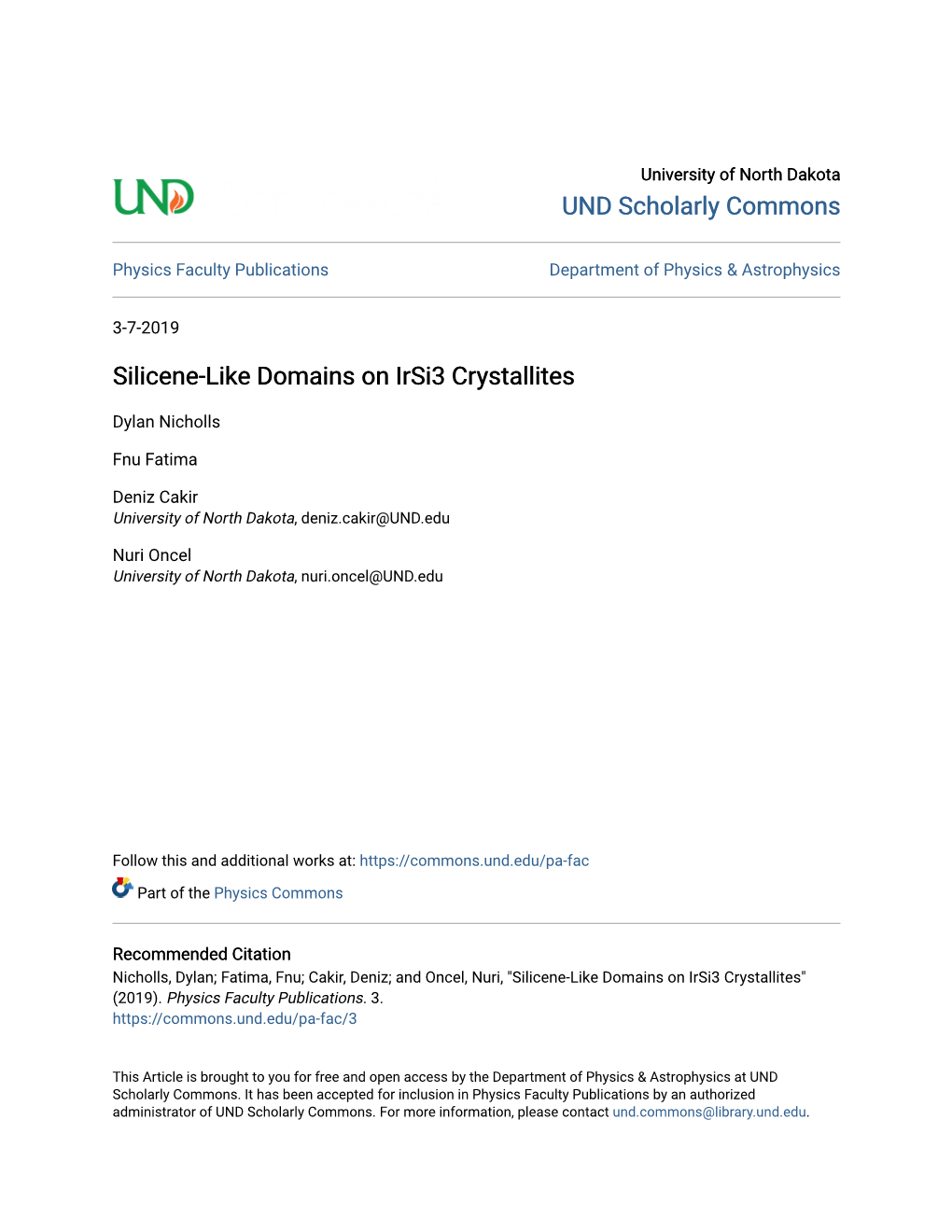
Load more
Recommended publications
-
Preserving the Q-Factors of Zno Nanoresonators Via Polar Surface Reconstruction
Home Search Collections Journals About Contact us My IOPscience Preserving the Q-factors of ZnO nanoresonators via polar surface reconstruction This article has been downloaded from IOPscience. Please scroll down to see the full text article. 2013 Nanotechnology 24 405705 (http://iopscience.iop.org/0957-4484/24/40/405705) View the table of contents for this issue, or go to the journal homepage for more Download details: IP Address: 128.197.57.225 The article was downloaded on 12/09/2013 at 20:41 Please note that terms and conditions apply. IOP PUBLISHING NANOTECHNOLOGY Nanotechnology 24 (2013) 405705 (8pp) doi:10.1088/0957-4484/24/40/405705 Preserving the Q-factors of ZnO nanoresonators via polar surface reconstruction Jin-Wu Jiang1, Harold S Park2,4 and Timon Rabczuk1,3,4 1 Institute of Structural Mechanics, Bauhaus-University Weimar, Marienstraße 15, D-99423 Weimar, Germany 2 Department of Mechanical Engineering, Boston University, Boston, MA 02215, USA 3 School of Civil, Environmental and Architectural Engineering, Korea University, Seoul, Korea E-mail: [email protected] and [email protected] Received 26 July 2013 Published 12 September 2013 Online at stacks.iop.org/Nano/24/405705 Abstract We perform molecular dynamics simulations to investigate the effect of polar surfaces on the quality (Q)-factors of zinc oxide (ZnO) nanowire-based nanoresonators. We find that the Q-factors in ZnO nanoresonators with free polar (0001) surfaces are about one order of magnitude higher than in nanoresonators that have been stabilized with reduced charges on the polar (0001) surfaces. From normal mode analysis, we show that the higher Q-factor is due to a shell-like reconstruction that occurs for the free polar surfaces. -
Atomic Force Microscopy Method Development for Surface Energy Analysis
University of Kentucky UKnowledge University of Kentucky Doctoral Dissertations Graduate School 2011 ATOMIC FORCE MICROSCOPY METHOD DEVELOPMENT FOR SURFACE ENERGY ANALYSIS Clare Aubrey Medendorp University of Kentucky, [email protected] Right click to open a feedback form in a new tab to let us know how this document benefits ou.y Recommended Citation Medendorp, Clare Aubrey, "ATOMIC FORCE MICROSCOPY METHOD DEVELOPMENT FOR SURFACE ENERGY ANALYSIS" (2011). University of Kentucky Doctoral Dissertations. 185. https://uknowledge.uky.edu/gradschool_diss/185 This Dissertation is brought to you for free and open access by the Graduate School at UKnowledge. It has been accepted for inclusion in University of Kentucky Doctoral Dissertations by an authorized administrator of UKnowledge. For more information, please contact [email protected]. ABSTRACT OF DISSERTATION Clare Aubrey Medendorp The Graduate School University of Kentucky 2011 ATOMIC FORCE MICROSCOPY METHOD DEVELOPMENT FOR SURFACE ENERGY ANALYSIS ABSTRACT OF DISSERTATION A dissertation submitted in partial fulfillment of the requirements for the degree of Doctor of Philosophy in the College of Pharmacy at the University of Kentucky By Clare Aubrey Medendorp Lexington, Kentucky Director: Dr. Tonglei Li, Professor of Pharmaceutical Sciences Lexington, Kentucky 2011 Copyright © Clare Aubrey Medendorp 2011 ABSTRACT OF DISSERTATION ATOMIC FORCE MICROSCOPY METHOD DEVELOPMENT FOR SURFACE ENERGY ANALYSIS The vast majority of pharmaceutical drug products are developed, manufactured, and delivered in the solid-state where the active pharmaceutical ingredient (API) is crystalline. With the potential to exist as polymorphs, salts, hydrates, solvates, and co- crystals, each with their own unique associated physicochemical properties, crystals and their forms directly influence bioavailability and manufacturability of the final drug product. -
Scanning Tunneling Microscopy
Scanning Tunneling Microscopy Nanoscience and Nanotechnology Laboratory course "Nanosynthesis, Nanosafety and Nanoanalytics" LAB2 Universität Siegen updated by Jiaqi Cai, April 26, 2019 Cover picture: www.nanosurf.com Experiment in room ENC B-0132 AG Experimentelle Nanophysik Prof. Carsten Busse, ENC-B 009 Jiaqi Cai, ENC-B 026 1 Contents 1 Introduction3 2 Surface Science4 2.1 2D crystallography........................4 2.2 Graphite..............................5 3 STM Basics6 3.1 Quantum mechanical tunneling.................7 3.2 Tunneling current.........................9 4 Experimental Setup 12 5 Experimental Instructions 13 5.1 Preparing and installing the STM tip.............. 13 5.2 Prepare and mount the sample................. 15 5.3 Approach............................. 16 5.4 Constant-current mode scan................... 17 5.5 Arachidic acid on graphite.................... 18 5.6 Data analysis........................... 20 6 Report 21 6.1 Exercise.............................. 21 6.2 Requirements........................... 21 2 1 Introduction In this lab course, you will learn about scanning tunneling microscopy (STM). For the invention of the STM, Heinrich Rohrer and Gerd Binnig received the Nobel prize for physics 1986 [1]. The STM operates by scanning a con- ductive tip across a conductive surface in a small distance and can resolve the topography with atomic resolution. It can also measure the electronic properties, for example the local density of states, and manipulate individ- ual atoms or molecules, which are adsorbed on the sample surface. Some examples are shown in Figure1. Figure 1: a) Graphene on Ir(111), Voltage U=0.1V; Current I=30nA [2], b) NbSe2 (showing atoms and a charge density wave (CDW)) [3], c) manipu- lation of Xe atoms on Ni(110). -
RUTHERFORD BACKSCATTERING SPECTROSCOPY( Dissertation 全文 )
MONOLAYER ANALYSIS USING HIGH-RESOLUTION Title RUTHERFORD BACKSCATTERING SPECTROSCOPY( Dissertation_全文 ) Author(s) Nakajima, Kaoru Citation 京都大学 Issue Date 2009-09-24 URL https://doi.org/10.14989/doctor.r12399 Right Type Thesis or Dissertation Textversion author Kyoto University MONOLAYER ANALYSIS USING HIGH-RESOLUTION RUTHERFORD BACKSCATTERING SPECTROSCOPY KAORU NAKAJIMA 2009 ACKNOWLEDGEMENTS I would like to express my deepest gratitude to Professor Kenji Kimura for the guidance, constructive suggestions, valuable discussions and continuous encouragement throughout this study. I would like to heartily appreciate Professor Motofumi Suzuki for his valuable advice and encouragement. I am grateful to Professors Michi-hiko Mannami, Yoshikazu Fujii and Yasufumi Susuki for offering the guidance and imparting the basic knowledge in this research field of at the beginning of this study. I am grateful to Professors Akio Itoh, Ikuji Tkagi, and Nobutsugu Imanishi, and Messrs. Kouji Yoshida and Keizou Norisawa, and the members of the Department of Nuclear Engineering and the Quantum Science and Engineering Center (QSEC) of Kyoto University for their kind help in the operation of the 4 MV Van de Graaff accelerator. I wish to thank many colleagues and students who have collaborated with me in this study. I was greatly helped by Dr. Kazumasa Narumi, Dr. Ming Zhao, Messrs. Kazuomi Ohshima, Yoshio Ooka, Atsushi Konishi, Yasutaka Okazaki, Noriyuki Hosaka, Yoshihiko Hano, Shinji Joumori, Kohei Kinoshita, Wataru Sakai, Yasutaka Okura, Shigetaka Hosoi, Akira I Fujiyoshi, and many other students in my experiments. Finally, I would like to sincerely thank my wife, Yae, my daughters, Chimomo, Chiori, Chihiro, and my parents, Yasuko and Shigeo for their reception of my research life and continuous encouragement. -
Introduction to Nanoparticles and Nanostructures
Introduction to Nanoparticles and Nanostructures www.nano4me.org © 2018 The Pennsylvania State University Intro to Nanoparticles and Nanostructures 1 Outline • Definition and Examples • Physical Properties - Size - Crystal Structure - Melting Point - Mechanical Strength • Optical Properties • Surface Plasmons • Quantum Confinement Effects • Electrical Properties • Health Concerns www.nano4me.org © 2018 The Pennsylvania State University Intro to Nanoparticles and Nanostructures 2 Definition of Nanoparticle • A structure with at least 1 dimension less than 1 µm. • Examples: – Sphere-like particles • Ag nanoparticles, buckyballs – Rod-like particles • Si & Ni nanowires – Tube-like particles • Carbon nanotubes • TiO2 nanotubes www.nano4me.org © 2018 The Pennsylvania State University Intro to Nanoparticles and Nanostructures 3 Size is a Material Property? The gold we know: The gold we are discovering: Material properties don’t Material properties (such as optical change with size Absorption, shown here) change with the - resistivity size of the gold nanoparticle. - melting point - optical absorption www.nano4me.org © 2018 The Pennsylvania State University Intro to Nanoparticles and Nanostructures 4 Outline • Definition and Examples • Physical Properties - Size - Crystal Structure - Melting Point - Mechanical Strength • Optical Properties • Electrical Properties • Health Concerns www.nano4me.org © 2018 The Pennsylvania State University Intro to Nanoparticles and Nanostructures 5 Unique Characteristics of Nanoparticles • Large surface to volume -
Surface Crystallography by X-Ray Diffraction
Surface Crystallography by X-ray Diffraction François Grey and Robert Feidenhans'l, Roskilde (Risø National Laboratory) It has been said that all science is ventional Miller indices hkl of a crystal either physics or stamp collecting. If so, plane can most easily be understood as then surface science has consisted the shortest reciprocal lattice vector mainly of stamp collecting for the last normal to it.) 20 years. The collection is a vast and Without further precaution, however, complex one, because crystal surfaces the diffuse X-ray scattering from the display a bewildering variety of struc bulk will swamp all but the most intense tures which depend delicately on, for fractional-order reflections. The solution example, the crystal temperature or the to this problem is to perform the diffrac amount of any adsorbed atoms on the tion experiment under grazing angles of surface. To extract some physics from incidence, thus limiting the X-ray pene this collection, scientists require reliable Fig. 1 — The diffraction geometry. The tration depth considerably. The diffrac models of the surface atomic geometry. monochromatic X-ray beam is incident at an tion geometry is shown in Fig. 1. In par Such models have proved difficult to angle αi to the surface. After being diffrac ticular, below a certain critical angle of obtain experimentally. ted through an angle 29 by the reconstruc incidence, typically a fraction of a The ideal surface of a crystal — the ted surface, the X-rays are detected at an degree, the X-rays are totally reflected surface obtained by simply terminating exit angle αf, to the surface, αi, and αf are typically of the order 0.5°. -
Molecular Precursors-Induced Surface Reconstruction at Graphene/Pt(111) Interfaces
Molecular Precursors-Induced Surface Reconstruction at Graphene/Pt(111) Interfaces Qian Wang, Rui Pang, and Xingqiang Shi* Department of Physics, South University of Science and Technology of China, Shenzhen 518055, China *E-mail: [email protected] Inspired by experimental observations of Pt(111) surfaces reconstruction at the Pt/graphene (Gr) interfaces with ordered vacancy networks in the outermost Pt layer, the mechanism of the surface reconstruction is investigated by van-der-Waals-corrected density functional theory in combination with particle-swarm optimization algorithm and ab initio atomistic thermodynamics. Our global structural search finds a more stable reconstructed (Rec) structure than that was reported before. With correction for vacancy formation energy, we demonstrate that the experimental observed surface reconstruction occurred at the earlier stages of graphene formation: 1) reconstruction occurred when C60 adsorption (before decomposition to form graphene) for C60 as a molecular precursor, or 2) reconstruction occurred when there were (partial) hydrogens retain in the adsorbed carbon structures for C2H4 and C60H30 as precursors. The reason can be attributed to that the energy gain, from the strengthened Pt-C bonding for C of C60 or for C with partial H, compensates the energy cost of formation surface vacancies and makes the reconstruction feasible, especially at elevated temperatures. In the Rec structure, two Pt-C covalent bonds are formed per unit cell, which have a great impact on the adsorbed Gr electronic structures. Key words: Pt/graphene interfaces, first principles calculation, reconstruction, vacancy formation energy, earlier stages of graphene. 1 I. INTRODUCTION The investigations of hybrid organic-metal interfaces are experiencing an explosive growth,1-4 especially for epitaxial growth of graphene (Gr) layers on metal surfaces. -
Single-Layer Tiox Reconstructions on Srtio3 (111): (√7×√7)R19.1°, T (√13 × √13)R13.9°, and Related Structures ⁎ Tassie K
Surface Science 675 (2018) 36–41 Contents lists available at ScienceDirect Surface Science journal homepage: www.elsevier.com/locate/susc Single-layer TiOx reconstructions on SrTiO3 (111): (√7×√7)R19.1°, T (√13 × √13)R13.9°, and related structures ⁎ Tassie K. Andersena, , Shuqiu Wangb, Martin R. Castellb, Dillon D. Fongc, Laurence D. Marksa a Department of Materials Science and Engineering, Northwestern University, Evanston, IL 60208, United States b Department of Materials, University of Oxford, Parks Road, Oxford OX1 3PH, UK c Materials Science Division, Argonne National Laboratory, Argonne, IL 60439, United States ARTICLE INFO ABSTRACT Keywords: The atomic structures of two reconstructions, (√7×√7)R19.1° and (√13 × √13)R13.9°, on the SrTiO3 (111) DFT surface were determined using a combination of density functional theory and scanning tunneling microscopy Polar oxide surfaces data and APW + lo density functional theory minimizations and simulations. These reconstructions belong to Surface structure the same structural family made up of an interconnected, single layer of edge-sharing TiO6 and TiO5[] octahedra. Oxide surfaces This family of reconstructions between 0.5 and 1.5 excess TiO representing the lowest-reported TiO coverages Surface defects 2, 2 for reconstructions on this surface. This family is found to include the previously-solved (2 × 2)a reconstruction. Strontium titanate They all follow a simple rule for surface composition, which serves as a tool for better understanding and predicting the structure of other reconstructions of arbitrary surface unit cell size on SrTiO3 (111). This re- construction family and the calculations of surface energies for different hypothesis structures also shed light on the structure of Schottky defects observed on these reconstructed SrTO3 (111) surfaces. -
Micro/Nanotribological Studies of Materials Using Atomic Force Microscopy Srinath R
Iowa State University Capstones, Theses and Graduate Theses and Dissertations Dissertations 2013 Micro/nanotribological studies of materials using Atomic force microscopy Srinath R. Kistampally Iowa State University Follow this and additional works at: https://lib.dr.iastate.edu/etd Part of the Mechanical Engineering Commons Recommended Citation Kistampally, Srinath R., "Micro/nanotribological studies of materials using Atomic force microscopy" (2013). Graduate Theses and Dissertations. 13280. https://lib.dr.iastate.edu/etd/13280 This Thesis is brought to you for free and open access by the Iowa State University Capstones, Theses and Dissertations at Iowa State University Digital Repository. It has been accepted for inclusion in Graduate Theses and Dissertations by an authorized administrator of Iowa State University Digital Repository. For more information, please contact [email protected]. Micro/nanotribological studies of materials using Atomic force microscopy by Srinath Kistampally A dissertation submitted to the graduate faculty in partial fulfillment of the requirements for the degree of MASTER OF SCIENCE Major: Mechanical Engineering Program of Study Committee: Sriram Sundararajan, Major Professor Pranav Shrotriya Scott Chumbley Iowa State University Ames, Iowa 2013 Copyright © Srinath Reddy Kistampally, 2013. All rights reserved. ii TABLE OF CONTENTS ACKNOWLEDGEMENTS ................................................................................... iii ABSTRACT .......................................................................................................... -
The Reconstruction of Nickel and Rhodium (001) Surfaces Upon Carbon, Nitrogen Or Oxygen Adsorptions
Surface Science 437 (1999) 18–28 www.elsevier.nl/locate/susc The reconstruction of nickel and rhodium (001) surfaces upon carbon, nitrogen or oxygen adsorptions Dario Alfe` 1, Stefano de Gironcoli, Stefano Baroni * SISSA — Scuola Internazionale Superiore di Studi Avanzati, and INFM — Instituto Nazionale di Fisica della Materia via Beirut 2–4, I-34014 Trieste, Italy Received 14 December 1998; accepted for publication 27 April 1999 Abstract Nickel and rhodium (001) surfaces display a similar (in terms of scanning tunneling microscopy images) clock reconstruction when half a monolayer of C–Ni, N–Ni or O–Rh is adsorbed; no reconstruction is observed instead for O–Ni. Adsorbate atoms sit at the center of the black squares of a chess-board, c(2×2), pattern and two different reconstructions are actually compatible with the observed STM images — showing a (2×2)p4g pattern — according to whether a rotation of the black or white squares occurs. We report on a first-principles study of the structure of X–Ni(001) and X–Rh(001) surfaces ( X=C, N, O) at half a monolayer coverage, performed using density-functional theory. Our findings are in agreement with all available experimental information and shed new light on the mechanisms responsible for the reconstructions. We show that the same substrate may display different reconstructions (or no reconstruction) upon adsorption of different atomic species, depending on the relative importance of the chemical and steric factors that determine the reconstruction. © 1999 Published by Elsevier Science B.V. All rights reserved. Keywords: Carbon, nitrogen and oxygen adsorption; Clock reconstruction; Nickel; Rhodium; Surface reconstruction; Density functional calculations 1. -
Excess Li-Ion Storage on Reconstructed Surfaces Of
Letter pubs.acs.org/NanoLett Excess Li-Ion Storage on Reconstructed Surfaces of Nanocrystals To Boost Battery Performance † † † † ‡ ‡ Yandong Duan, Bingkai Zhang, Jiaxin Zheng, Jiangtao Hu, Jianguo Wen, Dean J. Miller, § † † † † † ∥ † Pengfei Yan, Tongchao Liu, Hua Guo, Wen Li, Xiaohe Song, Zengqing Zhuo, , Chaokun Liu, † † ‡ ∥ † ⊥ § Hanting Tang, Rui Tan, Zonghai Chen, Yang Ren, Yuan Lin, Wanli Yang, Chong-Min Wang, # ‡ ‡ † Lin-Wang Wang, Jun Lu, Khalil Amine, and Feng Pan*, † School of Advanced Materials, Peking University, Shenzhen Graduate School, Shenzhen 518055, People’s Republic of China ‡ ∥ Center for Nanoscale Materials, X-ray Science Division, Argonne National Laboratory, Argonne, Illinois 60439, United States § Environmental Molecular Sciences Laboratory, Pacific Northwest National Laboratory, 902 Battelle Boulevard, Richland, Washington 99352, United States ⊥ # Advanced Light Source, Materials Science Division, Lawrence Berkeley National Laboratory, Berkeley, California 94720, United States *S Supporting Information ABSTRACT: Because of their enhanced kinetic properties, nanocrystallites have received much attention as potential electrode materials for energy storage. However, because of the large specific surface areas of nano- crystallites, they usually suffer from decreased energy density, cycling stability, and effective electrode capacity. In this work, we report a size-dependent excess capacity beyond theoretical value (170 mA h g−1) by introducing extra lithium storage at the reconstructed surface in nanosized LiFePO4 (LFP) -
Alternative Route to Silicene Synthesis Via Surface Reconstruction on H‑Mosi2 Crystallites
Letter pubs.acs.org/NanoLett Alternative Route to Silicene Synthesis via Surface Reconstruction on ‑ h MoSi2 Crystallites Cameron Volders, Ehsan Monazami, Gopalakrishnan Ramalingam, and Petra Reinke* Department of Materials Science and Engineering, University of Virginia, 395 McCormick Road, Charlottesville, Virginia 22904, United States ABSTRACT: Silicene is a two-dimensional material with a Dirac-type band structure and it is particularly attractive due to its potential for integration with Si-based technology. The primary focus has been to grow single silicene layers and understand how the electronic structure is affected by the substrate and the phase transition between low- and high-buckling configurations. Typically, silicene is synthesized by depositing monolayer amounts of silicon onto a heated Ag(111) surface; however, other growth substrates such as Ir(111) and ZrB2 have been studied recently. We present a novel route for silicene synthesis via a high- temperature surface reconstruction of hexagonal-MoSi2 nanocrystallites. The h-MoSi2 crystallites are formed by annealing of thin Mo-layers on Si(100)-(2 × 1) and their crystallographic orientation is controlled via an epitaxial relation with the Si-substrate. The (0001) plane of h-MoSi2 is comprised of Si-hexagons with a Mo atom residing in the center. Annealing above approximately 650 °C causes the (0001) plane to undergo a surface reconstruction process leaving a honeycomb pattern on the surface of these crystallites as shown by scanning tunneling microscopy. We define this surface layer as a silicene-like reconstruction (SLR), and a detailed geometric analysis of our structure yields a perfect match with the (√3 × √3)R30° silicene superstructure in a low- fi × buckled con guration (ABA̅).