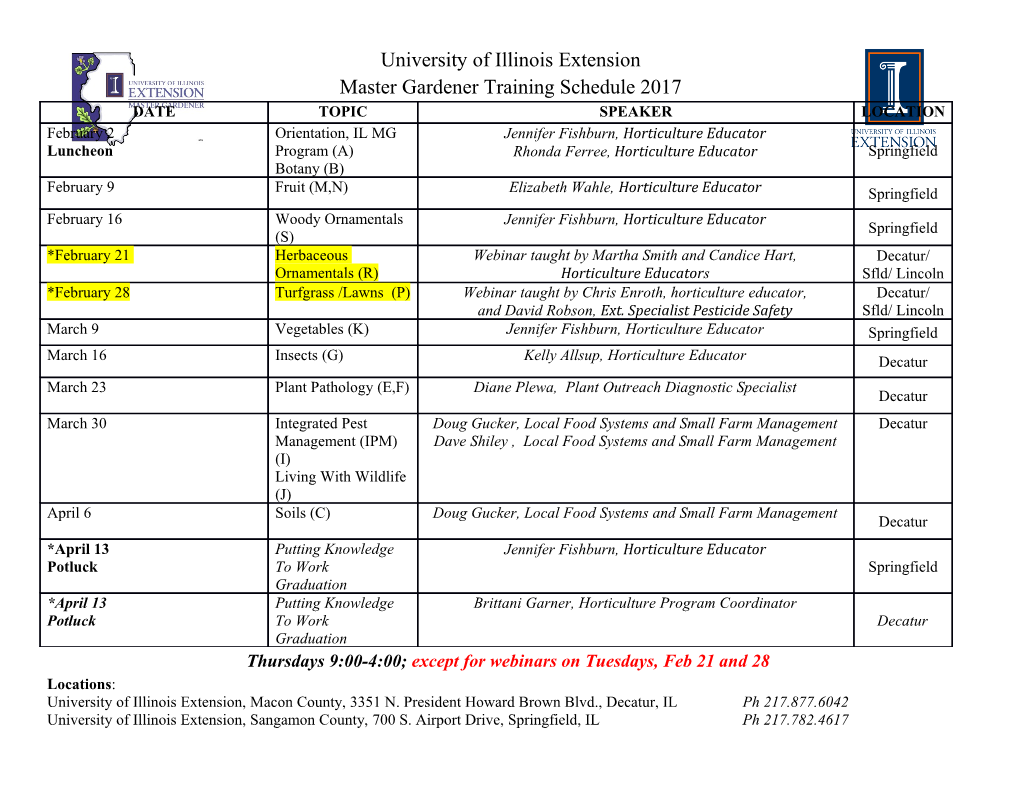
Observation of air showers with the IceAct 7 pixel demonstrator in coincidence with IceCube and IceTop von Erik Ganster Masterarbeit in Physik Vorgelegt der Fakultät für Mathematik, Informatik und Naturwissenschaften der Rheinisch-Westfälischen Technischen Hochschule Aachen im Oktober 2018 angefertigt am III. Physikalischen Institut B Univ.-Prof. Dr. Christopher Wiebusch revised version, January 2019 Betreuer Dr. Jan Auenberg III. Physikalisches Institut B RWTH Aachen Erstgutachter Univ.-Prof. Dr. Christopher Wiebusch III. Physikalisches Institut B RWTH Aachen Zweitgutachter Dr. Andreas Haungs Institute for Nuclear Physics Karlsruhe Institute of Technology iii iv Contents 1 Introduction1 2 Cosmic Rays3 2.1 Energy Spectrum . .3 2.2 Air Showers . .5 2.3 Cherenkov Light . .7 3 The IceCube Neutrino Observatory 11 3.1 The IceCube In-Ice Array . 12 3.2 The surface detector IceTop . 16 3.3 IceCube-Gen2 . 17 4 The IceAct Telescope 19 4.1 Mechanical structure/design . 21 4.2 Camera and Optics . 22 4.3 Silicon Photomultiplier (SiPM) . 25 4.4 The IceAct demonstrator at South Pole . 29 4.4.1 Camera . 30 4.4.2 Data Acquisition System . 32 4.4.3 Telescope Operation . 34 5 Coincident Data Taking with IceAct and IceCube 37 5.1 Connection between IceAct and IceCube . 37 5.2 Method of Time Synchronization . 39 5.3 Time Synchronization Results . 41 6 Analysis of Coincident Events 45 6.1 Analysis methods . 45 6.1.1 Software trigger . 45 6.1.2 Signal Extraction . 47 v Contents 6.2 Event Selection . 48 6.2.1 IceAct Event Selection . 48 6.2.2 IceTop Event Selection . 54 6.2.3 IceCube Event Selection . 56 6.3 IceAct Telescope Performance . 59 6.3.1 Observing Cosmic Rays . 64 6.3.2 Energy threshold of the IceAct Telescope . 72 6.4 Correlation between IceAct and IceCube/IceTop . 74 7 Summary and Outlook 77 A Appendix 81 Bibliography 83 List of Figures 91 List of Tables 95 vi 1 Introduction For more than a decade scientists all over the world have searched for the origin of cosmic rays and tried to explain their acceleration mechanisms leading to energies of 1020 eV. The IceCube Neutrino Observatory is a 1 km3 lage neutrino telescope melted deep in the glacial ice at the geographic South Pole. It was built to search for very high energetic neutrinos of astrophysical origin. The identication of their sources could answer the question for the sources of high energy cosmic rays, since they are believed to be the same. An air shower surface detector called IceTop is located above the IceCube in- ice array. It measures cosmic rays and can be used as a veto for cosmic ray induced muons and neutrinos that are the main background in IceCube's search for astrophysical neutrinos. A larger air shower detector on the surface is part of the Gen-2 extension of Ice- Cube. These surface detector should improve the veto capabilities of IceTop as well as the investigation of cosmic rays. One idea is the use of an array of small imaging air Cherenkov telescopes called IceAct. By observing an independent component of air showers, Cherenkov light, with a low energy threshold they could improve IceTop's com- position measurements and veto cosmic rays by using the whole atmosphere in their eld of view as an active volume. In order to demonstrate the capabilities of such an air Cherenkov telescope even in the harsh environments at South Pole, an IceAct telescope demonstrator was installed in December 2015. After a brief introduction to cosmic rays and the principle of Cherenkov radiation an introduction to the IceCube Neutrino Observatory is given. The concept of Ice- Act telescopes is introduced followed by a chapter on how data is taken in coincidence with IceAct, IceCube and IceTop. The focus of this thesis is the analysis of coincident events recorded with all three detectors in 2016 proong a successful operation of an air Cherenkov telescope at South Pole and its dierent capabilities: vetoing cosmic ray air showers, composition measurements together with IceTop and energy calibration for IceTop. Parts of this thesis will be published in a paper on the design and performance of the rst IceAct demonstrator at South Pole. 1 1 Introduction 2 2 Cosmic Rays Cosmic ray is a collective term for charged particles or nuclei that traverse through the interstellar medium, reach Earth and enter atmosphere. During balloon ights in 1912 Victor Hess observed a faster discharge of electroscopes with increasing altitude [1]. He correctly concluded that the ionization of air must increase with altitude causing the electroscopes to lose their charge faster. This ionization is caused by cosmic rays hitting the atmosphere and producing secondary charged particles in extensive air showers. The origin of cosmic rays reaching energies of more than 1020 eV (gure 2.1) is still unknown, as well as mechanisms accelerating particles to these energies or their sources. This chapter will give an introduction to cosmic rays and their well measured energy spectrum. It describes how air showers evolve in the atmosphere and what eect is used to measure these air showers at highest energies. 2.1 Energy Spectrum The dierential energy spectrum of cosmic rays is shown in gure 2.1. Measurements of dierent experiments are combined to cover an energy range from 1013 eV to 1020 eV. The dierential ux, or number of particles, of cosmic rays is given in units of 1=(GeV m2 s sr). The spectrum follows a power law of the form: dN / Eγ : (2.1) dE To point out three important features of the spectrum it is often multiplied by a factor of Ex. In gure 2.1, it is multiplied with a factor of E2:6 and its main features (knee, 2nd knee and ankle) are marked. Direct measurements of cosmic rays are feasible up to energies of about 100 TeV. For higher energies large detection areas are required to collect a suitable number of events in a reasonable time. The power law behavior causes the rate to heavily decrease from more than 1000 particles per second and square meter at GeV energies to about one particle per square meter and year at PeV.[2] 3 2 Cosmic Rays Figure 2.1: Energy spectrum of cosmic rays. Flux measurements of dierent experi- ments are shown. The spectrum is multiplied by E2:6 to point out its features: knee, 2nd knee and ankle. Taken from [3]. A steepening of the spectrum occurring between 1015 eV to 1016 eV is called knee (gure 2.1). At the knee the spectral index γ of equation (2.1) changes from ≈ −2:7 to ≈ −3:1, a softening of the spectrum. Energy and ux measurements of cosmic rays at the knee and beyond rely on indirect measurements of secondary particles produced in air showers (section 2.2) by ground based instruments.[2, 4] A second, further softening of the spectrum at about 4 × 1017 eV is called 2nd knee. At about 4 × 1018 eV the spectrum recovers to a spectral index of ≈ −2:7 again as can easily be seen in gure 2.1. This transition is called ankle. Event rates lower than one particle per square kilometer and century above 100 EeV requires huge detectors for measurements. The worlds largest detector for cosmic rays is the Pierre Auger Cosmic Ray Observatory in the Province of Mendoza, Argentina, covering an area of ≈ 3000 km2 [5].[2] 4 2.2 Air Showers Dierent models have been evolved to explain the knee and the ankle in the cosmic rays' energy spectrum. Common explanations uses dierent acceleration mechanisms and origins to describe these features. Knee and 2nd is associated with an upper limit of acceleration in galactic sources like supernova remnants and dierent compositions from light particles to heavy nuclei. The ankle, on the other hand, is the onset of an extra galactic component with a harder spectrum compared to galactic sources.[2, 6] An interaction of very high energetic cosmic rays with photons from the cosmic mi- crowave background (CMB) called GZK-cuto would lead to a hard cuto in the en- ergy spectrum at about 6 × 1019 eV. The GZK-cuto is driven by production of a ∆+- resonance: p + γ ! ∆+ ! p + π0 ! n + π+ : It was introduced and rst calculated by K. Greisen, G. T. Zatsepin and V. A. Kuzmin in 1966 giving it the name GZK-cuto shortly after the CMB was discovered by A. Penzias and R. Wilson [79]. 2.2 Air Showers Primaries of the cosmic rays will produce so called air showers when they hit Earth's atmosphere and interact with air nuclei. Such air showers can be divided into two categories (or parts): electromagnetic and hadronic showers. A simple model for the description of processes in electromagnetic showers was introduced by W. Heitler in 1954 and can be expanded to hadronic cascades. The model cannot replace fully detailed simulations of the processes inside an air shower, especially for hadronic ones, but it describes the most important features fairly well. The Heitler model for electromagnetic and its adapted version for hadronic showers is shown in gure 2.2 and shall be discussed in more detail.[10, 11] The left side of gure 2.2 shows an electromagnetic shower induced by a primary high energy photon. In a rst step the photon produces an electron-positron pair. Each elec- tron and positron will radiate a single photon by bremsstrahlung after traveling a certain distance in the atmosphere. In both cases the energy is assumed to be equally divided between both outgoing particles. Both processes, pair production and bremsstrahlung repeats several times and form an electromagnetic cascade of high energetic particles.
Details
-
File Typepdf
-
Upload Time-
-
Content LanguagesEnglish
-
Upload UserAnonymous/Not logged-in
-
File Pages103 Page
-
File Size-