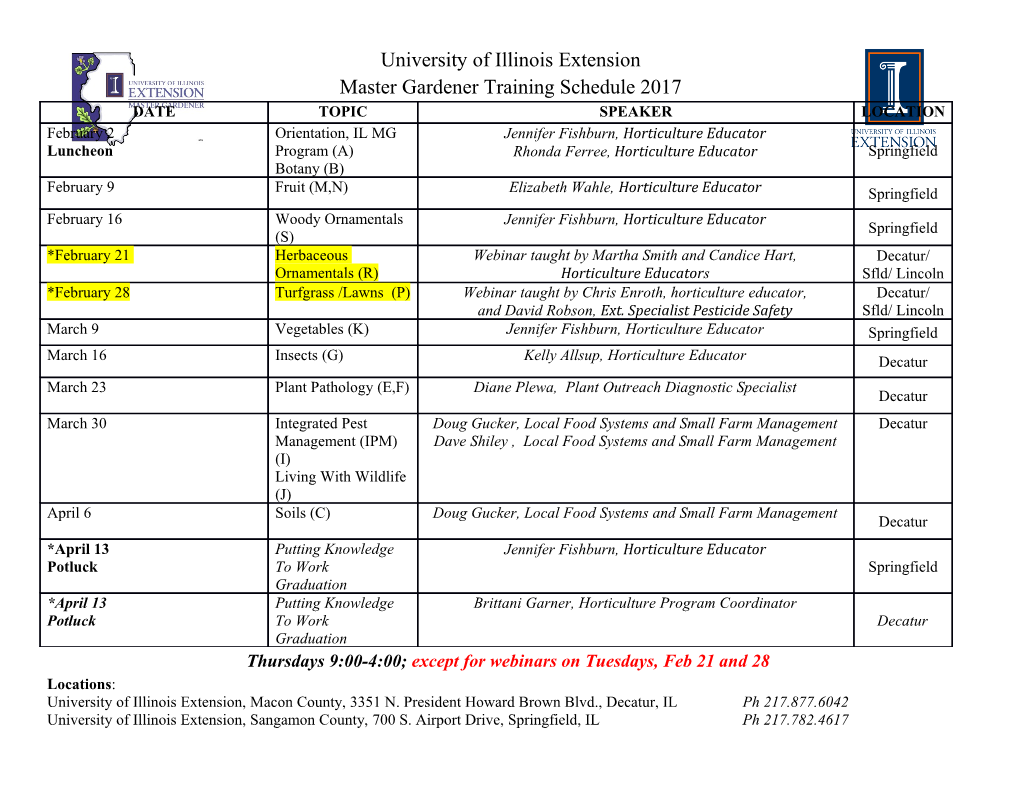
Atomic, molecular and optical physics applications of longitudinally coherent and narrow bandwidth Free-Electron Lasers Carlo Callegaria,∗, Alexei N. Grum-Grzhimailob, Kenichi L. Ishikawae, Kevin C. Princea,∗, Giuseppe Sansonec, Kiyoshi Uedad aElettra Sincrotrone Trieste, 34039 Basovizza, Italy bLomonosov Moscow State University, Moscow 119991, Russia cUniversity of Freiburg, Physikalisches Institut, Albert-Ludwigs-Universität Freiburg, 79106 Freiburg, Germany dInstitute of Multidisciplinary Research for Advanced Materials, Tohoku University, Sendai 980-8577, Japan eGraduate School of Engineering, The University of Tokyo, 7-3-1 Hongo, and Bunkyo-ku, Tokyo 113-8656, and Research Institute for Photon Science and Laser Technology, The University of Tokyo, 7-3-1 Hongo, Bunkyo-ku, Tokyo 113-0033, Japan Abstract Short wavelength Free-Electron Lasers (FELs) are the newest light sources available to scientists to probe a wide range of phenomena, with chemical, physical and biological applications, using soft and hard X-rays. These sources include the currently most powerful light sources in the world (hard X-ray sources) and are characterised by extremely high powers and high transverse coherence, but the first FELs had reduced longitudinal coherence. Now it is possible to achieve good longitudinal coherence (narrow bandwidth in the frequency domain) and here we discuss and illustrate a range of experiments utilising this property, and their underlying physics. The primary applications are those which require high resolution (for example resonant experiments), or temporal coherence (for example coherent control experiments). The currently available light sources extend the vast range of laboratory laser techniques to short wavelengths. Keywords: FELs, AMO, short-wavelength coherence. 2010 MSC: 78A60, 81V45 PACS: 41.60.Cr Contents 1 Introduction 3 1.1 Historical background of FEL sources . 3 1.2 Introduction to Atomic, Molecular and Optical science with FELs . 5 1.3 Role of transverse and longitudinal coherence. 7 2 Methods 7 2.1 Seeded soft X-ray and XUV FELs. 7 2.2 Self-seeded soft and hard X-ray FELs. 9 2.3 Alternative light sources. 10 3 Optical experiments with FELs 10 3.1 SASE FELs as pumps for fully coherent X-ray FELs . 10 arXiv:2008.11024v1 [physics.atom-ph] 25 Aug 2020 3.2 Superfluorescence and related phenomena . 11 ∗Corresponding author Email addresses: [email protected] (Carlo Callegari), [email protected] (Alexei N. Grum-Grzhimailo), [email protected] (Kenichi L. Ishikawa), [email protected] (Kevin C. Prince), [email protected] (Giuseppe Sansone), [email protected] (Kiyoshi Ueda) Preprint submitted to Physics Reports August 26, 2020 4 Few photon, single ionization 12 4.1 Brief introduction to photoionization . 12 4.2 One-colour, two-photon ionization . 13 4.3 XUV/X-ray + Near Infrared ionization and Circular Dichroism . 14 4.4 Orbital Angular Momentum . 16 4.5 Towards two-dimensional XUV spectroscopy . 16 4.6 Coherent control of one-colour ionization via biharmonic ionization . 17 4.7 Experimental determination of optical phase . 19 4.8 Photoemission time delay . 19 4.9 Generation and characterization of attosecond pulse trains . 20 5 Few-photon sequential multiple ionization 24 5.1 Early studies . 24 5.2 Complete experiments on Ne . 24 5.3 The role of autoionizing resonances in double ionization . 25 5.4 Single-photon Laser Enabled Auger Decay . 26 5.5 Two-photon excitation leading to interatomic Coulombic decay . 27 5.6 Multiphoton XUV + Near Infrared ionization and Circular Dichoism . 29 5.7 Two-photon, double core ionization . 29 6 Multi-photon and multiple resonant excitation 30 6.1 Two-photon excitation of two electrons in atoms: Early studies . 30 6.2 Two-photon excitation of two electrons in atoms: Narrow-band studies . 31 6.3 Two-photon excitation of two electrons in dimers . 31 6.4 Multi-photon, site-specific, multiple excitation of clusters and droplets . 32 7 Pump-probe studies 32 7.1 The dynamics of photo-excited thymine . 33 7.2 Photo-excited acetylacetone . 33 7.3 Isomerization of thiophenone . 34 7.4 Dynamics of photo-excited He droplets . 35 8 Theoretical approaches 35 8.1 Amplitudes and description of physical quantities . 35 8.2 Time-dependent Schrödinger equation (TDSE) . 36 8.3 Solution of the TDSE on the space-time grid . 37 8.4 Expansion methods . 37 8.5 Perturbation theory . 39 8.6 Strong-field-type approximations . 40 8.7 Density matrix approach . 40 8.8 Real-time ab initio simulations: Time-dependent multiconfiguration self-consistent-field method . 41 8.9 Theoretical proposals for XFEL experiments . 43 9 Perspectives 45 10 Appendix: List of symbols 47 11 Acknowledgements 48 2 1. Introduction Free-Electron Lasers are modern light sources which use high energy beams of electrons moving in vacuum to generate radiation. Although there are many such sources producing infrared light, in this paper we focus on those producing ionizing radiation, that is, wavelengths from the Extreme Ultraviolet (XUV; 124–10 nm) to soft and hard X-rays (<10 nm). 1.1. Historical background of FEL sources The interaction of light with matter is governed by the Einstein coefficients, which describe the spontaneous emission, absorption and stimulated emission of light. Einstein’s recognition of the importance of stimulated emission was the basis for the invention (much later) of the maser (Gordon et al., 1954, 1955) and laser (Schawlow and Townes, 1958; Maiman, 1960). In atoms, molecules, and solids the electrons involved in the lasing process are all bound in specific orbitals or bands, that is, they reside in a strong electromagnetic field. The wavelengths which such lasers can emit is limited by the quantum transitions which are possible, so that it is not possible to tune over wide ranges. Long ago it was recognised that stimulated emission may occur in free electrons, that is, electrons moving in vacuum. The word “free” is perhaps slightly misleading, as an electron moving in field-free space cannot be stimulated to emit radiation. Rather, the electron is free in the sense that it is not confined to an atom or molecule but moves in an external field. Microwave devices, such as klystrons, are mostly based on electric fields, whereas short-wavelength devices are generally based on relativistic particle accelerator technology, where high-energy electrons are deflected, focused, and guided by magnetic fields. Since the electron energy and magnetic fields can be tuned continuously, the idea of using light from free electrons travelling through a periodic magnetic structure (Ginzburg, 1947; Motz, 1951) offered the possibility of a light source with continuously tunable wavelength (Motz et al., 1953). Let us note that because of the methods used to produce and accelerate the electrons, the latter are not uniformly distributed, but rather are grouped in packets (“bunches”). A strong distinction must be made between microwave emission at one end (where the bunch size can be externally controlled, and be shorter than the radiation wavelength) and X-rays at the other end (where the bunch size is much longer than the radiation wavelength). In the latter case one generally ignores the finite length of the bunch, and analyzes the periodic grouping of the electrons within the bunch. This grouping, which grows as part of the amplification process, is termed “microbunching” (Yu, 1984), and the short form “bunching”, is a confusing but accepted terminology. Motz (1951) recognized the importance of pre-bunching to make the radiation coherent, and even analyzed the case of an electron moving through an electromagnetic wave traveling in the opposite direction, but did not consider the process of stimulated emission and the associated bunching growth. This was later done by Madey (1971), who is generally credited as the father of the Free-Electron Laser; an analysis of the formal equivalence of the spontaneous and stimulated emission from electrons in atoms and that from quasifree electrons was later done by Friedman et al. (1988). There exist several reviews of the history of Free-Electron Lasers: Colson and Sessler (1985) trace the evolution of free-electron sources back to the 1940s, from non-relativistic electron tubes, and list a number of schemes suitable for long-wavelength operation (down to ∼ 5000 Å). Madey (2014) gives a personal account of how short-wavelength free-electron lasers are the result of a cross fertilization between the fields of electron tubes, masers/lasers, and particle accelerators, and borrow fundamental concepts from all these fields. The considered electron energies varied over a broad range, hundreds of keV to hundreds of MeV; modern FELs all utilise electron beams of GeV energies, and are thus highly relativistic devices (we recall that the rest energy of the electron is 0.511 MeV); a non-relativistic precursor – the “ubitron” – was built by Phillips (1960, 1988). Just as for optical lasers, long-wavelength FELs can be classified as oscillators or amplifiers, depending on the presence or absence of a resonant cavity (Colson and Sessler, 1985). Early FELs were conceived as low-gain ampli- fiers (Madey, 1971; Madey et al., 1973) and later as oscillators, for wavelengths where mirrors were available. The efficiency and tunability were seen as promising characteristics, and applied research on single-pass FELs was carried out, for example by the United States Office of Naval Research (Roberson and Sprangle, 1989a). The coherence properties of FELs were immediately recognised as important, and at long wavelength longitudinal coherence was not difficult to attain. There was clearly great interest in FELs as sources for short wavelength ranges, where no convenient alternatives were available; high harmonic generation from FELs operating as single-pass am- plifiers was recognized as a means of overcoming the limitations of mirrors in the UV region and beyond (Colson, 1981; Colson et al., 1985). Kondratenko and Saldin (1979, 1980) proposed a single-pass amplifier (in the infrared) 3 starting from noise, and Murphy and Pellegrini (1985) extended the concept to soft X-rays.
Details
-
File Typepdf
-
Upload Time-
-
Content LanguagesEnglish
-
Upload UserAnonymous/Not logged-in
-
File Pages69 Page
-
File Size-