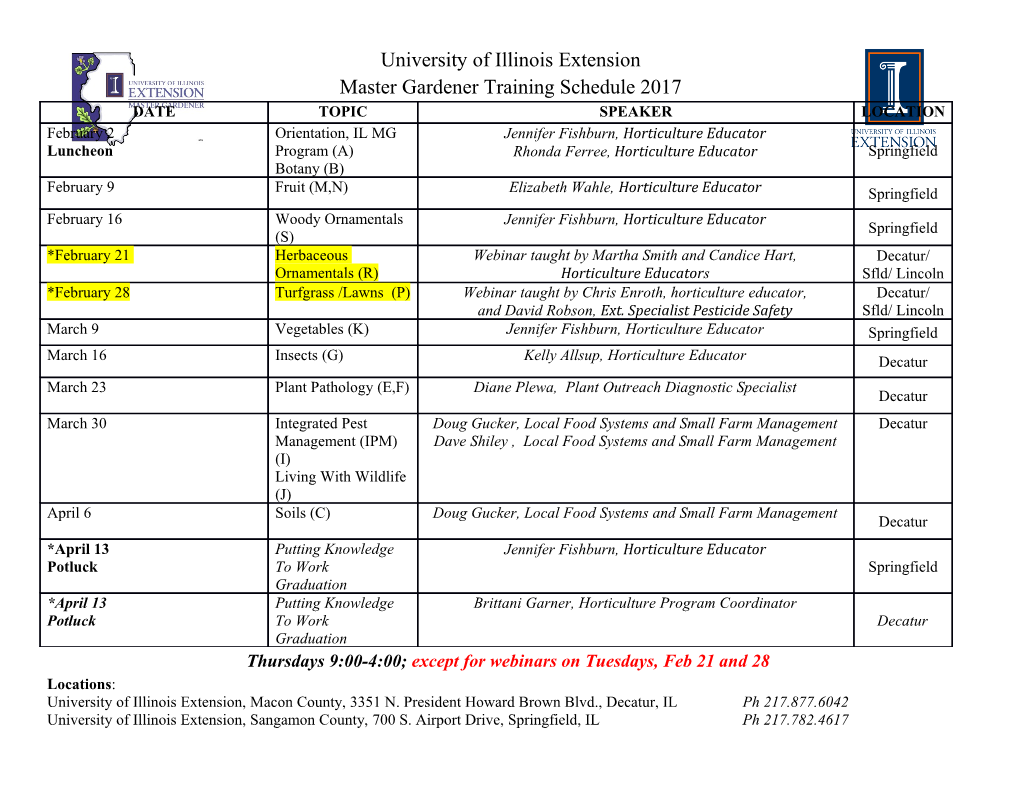
1. Group actions and other topics in group theory October 11, 2014 The main topics considered here are group actions, the Sylow theorems, semi-direct products, nilpotent and solvable groups, and simple groups. See Preliminary remarks for some of the notation used here, especially regarding general linear groups. Some further notation: [n] denotes the set of the first n natural numbers 1; 2; :::; n. Pk[n] denotes the set of k-element subsets of [n]. 1 Group actions 1.1 Definition of a group action or G-set Let G be a group, with identity element e.A left G-set is a set X equipped with a map θ : G × X−!X satisfying (i) θ(gh; x) = θ(g; θ(h; x)) for all g; h 2 G and all x 2 X, and (ii) θ(e; x) = x for all x 2 X. Usually we write either g · x or simply juxtaposition gx for θ(g; x); in the latter notation conditions (i) and (ii) become (gh)x = g(hx) and ex = x. We also call this data a group action, or say that \G acts on X" (on the left). Similarly a right G-set is a set X equipped with a map θ : X × G−!X satisfying (in the evident juxtaposition notation) x(gh) = (xg)h and xe = x. Of course the distinction between left and right G-actions does not depend on whether we write the domain of θ as G × X or X × G. The distinction is that in a left action gh acts by h first, then g, whereas in a right action g acts first, then h. Notice that up to this point, we haven't even used the existence of inverses, so exactly the same definition makes sense for left and right monoid actions. We will make little use of monoid actions, however. One immediate advantage of the existence of inverses is that any right action can be converted to a left action by setting g · x = xg−1; similarly any left action can be converted to a right action. Nevertheless it is important to pay close attention to which side the group is acting on. If the side is not specified we always mean a left action (an arbitrary choice on my part!). Any statement about left actions has a parallel statement for right actions; we leave it to the reader to make the translation. 1.2 Some fundamental terminology Let X; Y be (left) G-sets. A map φ : X−!Y is a G-map or a G-equivariant map if φ(gx) = gφ(x) for all g 2 G, x 2 X. Then G-sets and G-maps form a category that we will denote 1 G-Set. The fixed-point set XG is defined by XG = fx 2 X : gx = x 8g 2 Gg. This defines a functor G-set −! Set in the evident way. The isotropy group Gx of a point x 2 X is defined by Gx = fg 2 G : gx = xg; clearly Gx is a subgroup. Thus the fixed-points are the points x with Gx = G. The action is trivial if every point is a fixed point. At the opposite extreme, the action is free if Gx = feg for all x 2 X. Define an equivalence relation on X by x ∼ y if there exists g 2 G such that gx = y.A equivalence class is called an orbit, usually denoted O. The orbit determined by a particular x 2 X is denoted Ox or Gx. The set of all orbits of a left action is denoted GnX; the set of orbits of a right action is denoted X=G. This notational distinction is important because we will often have groups acting on the left and the right of the same set X. The action is transitive if there is only one orbit. In other words, for all x; y 2 X there exists a g such that gx = y. Transitive actions will be discussed in more detail in a later section. If O ⊂ X is an orbit and x 2 O, then the map G−!X given by g 7! gx factors through =∼ a G-bijection G=Gx −! O = Gx. Hence [G : Gx] = jGxj (this is true even when the sets in question are infinite, but we have in mind here the finite case). Finally, we recall a simple but very powerful counting formula. Suppose the group G acts on the finite set X. Then X X jXj = jOj = [G : Gx]; O x2GnX where the first sum is over the orbits O of the action and the second sum means, in a mildly abusive notation, that we are taking a fixed representative x of each orbit. This choice of x 2 O is arbitrary, but the sum is nevertheless well-defined since [G : Gx] = jOj is independent of the choice. 2 Examples 1. The symmetric group Sn acts on the left of [n] := f1; 2; :::; ng by permutions. The action is transitive, with the isotropy group of any point isomorphic to Sn−1. More generally, if X is any set, we let P erm X denote the group of bijections X−!X. Then by construction P erm X acts on the left of X by σ · x = σ(x). It is a left action because a composition σ ◦ τ acts by τ first, then σ. 2. If G is any group, H any subgroup, then the left translation action of H on G is defined by h · g = hg for h 2 H, g 2 G. The right translation action is given by g · h = gh. These are both free actions. The orbit space HnG of the left action is by definition the set of right cosets Hg, while the orbit space G=H of the right action consists of the left cosets gH. If G is finite, then (since every orbit has size jHj) the counting formula just says that jGj = jHj · [G : H]. 2 3. If G is any group, the left conjugation action of G on itself is given by g · x = gxg−1. Similarly right conjugation is defined by x · g = g−1xg. The fixed-point set of either action is the center C(G)(Z(G) is another common notation for the center). The orbits are the conjugacy classes of G. The isotropy group of x is CGx, the centralizer of x in G. In this case the counting formula yields the class equation. To state it we need a notation for conjugacy classes, and|sadly|we have already assigned the letter \C" to centers and centralizers. I will use the non-standard notation κ(x) to mean the conjugacy class of x, and Conj G to mean the set of conjugacy classes. We then have X X jGj = jκ(x)j = [G : CGx]: x2Conj G x2Conj G Once again the notation has the obvious interpretation: We are choosing one x from each conjugacy class, and the choice doesn't matter. 4. If G is any group, let S(G) denote the set of subgroups of G. Then G acts on S(G) by left conjugation: g · H = gHg−1 (there is also a right conjugation, of course). The fixed-points are the normal subgroups. The orbits are conjugacy classes of subgroups. The isotropy group of H is the normalizer NGH of H in G. 5. Let X; Y be sets and let F (X; Y ) denote the set of functions X−!Y . If Y is a left G-set, we get a left G-action on F (X; Y ) by (g · φ)(x) = g(φ(x)). If X is a left G-set, we get a right G-action on F (X; Y ) by (φ · g)(x) = φ(gx). Note carefully that this is a right action. However, we can always convert it to a left action by (g ? φ)(x) = φ(g−1x). If both X and Y are G-sets, we can get a combined left action of G on F (X; Y ) by (g · φ)(x) = gφ(g−1x). The fixed-point set of the \combined" left action gφg−1 is the subset of G-equivariant maps X−!Y , as is easily checked. n 6. Let X be a set, X the n-fold Cartesian product of X with itself. Then Sn acts on Xn by permuting the coordinates. This is a right action, given explicitly by (x1; :::; xn) · σ = (xσ(1); :::xσ(n)): One could check directly that this is a right action, but easier is to note that Xn = F ([n];X), where Sn acts on the domain, on the left. So this is a special case of the previous example. The fixed-point set is the diagonal subset of all (x; x; :::; x). Contemplation of the orbits and isotropy groups is left to the reader. 7. Projective spaces. The purpose of this example is twofold. First of all, projective spaces are ubiquitous in topology and geometry|including especially algebraic geometry, which leads me to discuss them in an algebra course. Second, in \nature" we are often first confronted not with a group action or even a group, but with a set (or topological space, etc.) X that may secretly be equipped with a useful group action and/or realization as the orbit set of a group action. It's important to be able to recognize such structures. Let F be a field, and let V be a finite dimensional vector space over F . (In fact the finite-dimensionality isn't necessary, but I prefer to avoid distractions.) The projective space P(V ) is the set of lines through the origin in V .
Details
-
File Typepdf
-
Upload Time-
-
Content LanguagesEnglish
-
Upload UserAnonymous/Not logged-in
-
File Pages31 Page
-
File Size-