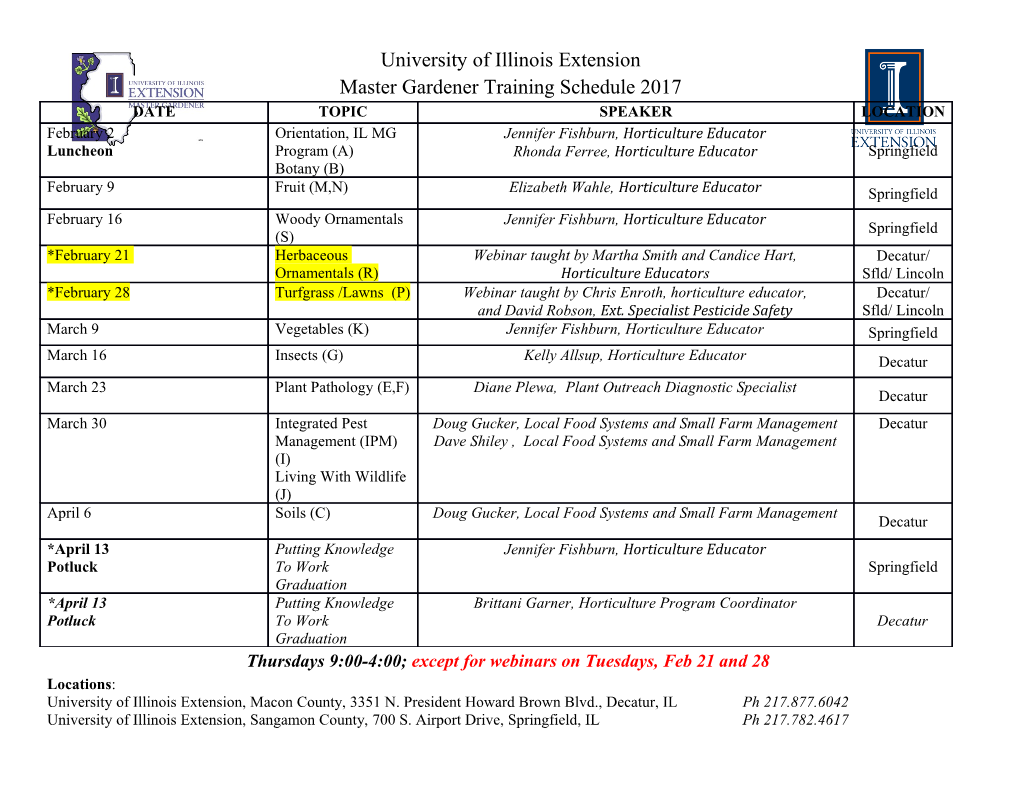
12 The Malverns, Cherry Orchard Lane, Salisbury SP2 7JG, U.K. Telephone: +44(0)1722 414282 Facsimile: +44(0)1722 414288 email: [email protected] website: www.techset.co.uk Use this as the cover page to fax corrections to your chapter Date: TO: Paul Beaney fax: 44(0) 1722 414288 email: [email protected] FROM: ........................................................... BOOK TITLE: Sigel: Metal Ions in Biological Systems, Volume 43 (ISBN 0-0000-0000-0) CHAPTER NUMBER: 07 Author: Please list page numbers of pages requiring correction, and record answers to queries and any special instructions here: Author: Please indicate the preferred mailing address for your complimentary copy of the finished book and special promotions or discounts reserved for Dekker authors. International Publishing Services with offices in Salisbury, London & Chennai Registered in England, no. 2420700 0000-0-Sigel-ch07_R1_121004 1 2 3 4 5 7 6 7 8 Iron, Phytoplankton Growth, 9 10 and the Carbon Cycle 11 12 13 14 Joseph H. Street and Adina Paytan 15 Department of Geological and Environmental Sciences, Braun Hall, 16 Stanford University, Stanford, California, USA 17 18 19 20 21 1. Iron, an Essential Nutrient for Marine Organisms 154 22 1.1. Iron Function in Cells 154 23 1.2. Cellular Uptake Mechanisms 155 24 1.3. Chemical and Physical Limits on Uptake Rates 156 25 1.4. Expression of Stress 157 26 2. Iron Chemistry in Seawater 158 27 2.1. Chemical Forms 158 28 2.2. Speciation and Redox Chemistry 159 29 2.3. Interaction with Organic Compounds 159 30 31 3. Iron Distribution and Cycling in the Ocean 160 32 3.1. External Iron Sources 160 33 3.1.1. Atmospheric Dust Deposition 160 34 3.1.2. River Input 162 35 3.1.3. Hydrothermal Sources 163 36 3.1.4. Mobilization in Sediments 163 37 3.2. Iron Cycling in the Ocean 164 38 3.2.1. Upwelling and Advection 164 39 3.2.2. Biological Cycling, Export, and Regeneration 165 40 3.2.3. Particle Scavenging 166 41 3.3. Patterns in the Distribution of Iron in the Ocean 166 42 3.3.1. Dissolved Iron in the Open Ocean 167 43 153 44 0000-0-Sigel-ch07_R1_121004 154 Street and Paytan 45 3.3.2. Dissolved Iron in the Coastal Ocean, Shallow 46 Seas, and Semi-enclosed Basins 169 47 3.3.3. Particulate Iron 169 48 3.3.4. Suboxic/Anoxic Zones 169 49 4. Iron Limitation of Marine Primary Productivity and Control on 50 Ecosystem Structure 170 51 4.1. Evidence for the Role of Iron in Regulating Productivity 52 in High Nutrient Low Chlorophyll Regions 170 53 4.1.1. Bottle Incubation Experiments 171 54 4.1.2. Mesoscale Enrichment Experiments 173 55 4.1.3. Evidence of Iron Limitation from Other Regions 176 56 4.2. Interaction of Iron with Other Limiting Factors 177 57 4.3. Carbon Export and Iron Fertilization 180 58 5. The Role of Iron in Regulating Atmospheric CO2 180 59 5.1. The Iron Hypothesis 180 60 5.2. Changes in Dust Input and Productivity in Glacial Periods 181 61 5.3. Consequences for Atmospheric CO2 and Global Climate 182 62 6. Summary and Conclusions 183 63 64 Acknowledgments 184 65 Abbreviations 184 66 References 185 67 68 69 70 71 72 1. IRON, AN ESSENTIAL NUTRIENT FOR MARINE ORGANISMS 73 74 1.1. Iron Function in Cells 75 Iron is an essential nutrient supporting the growth and metabolism of marine 76 organisms. Iron-bearing molecules are involved in photosynthetic and respiratory 77 electron transport, nitrate and nitrite reduction, N2-fixation, sulfate reduction, 78 and, as enzyme components, in the detoxification of reactive oxygen species 2 79 such as O2 and H2O2 [1]. 80 Relative to other nutrients, iron is disproportionately utilized in cell metab- 81 olism over cell structure. As a result, cellular Fe : C ratios tend to vary with Fe 82 availability (and, by extension, metabolic rate), in contrast to the more or less 83 fixed ratios of C : N : P measured in marine phytoplankton [2,3]. Fe : C ratios 84 measured in pelagic phytoplankton and marine particulate matter range from 85 1 : 3000 to 1 : 500,000 [3–7]. This variation in cellular Fe content is intrinsic to 86 different classes of phytoplankton as has been shown in culture experiments 87 [8]. In particular, because iron is present in the catalytic centers of nitrate- and 88 nitrite-reductase enzymes, in reducing equivalent molecules (NADPH, ferredoxin) and in the N2-fixing enzyme nitrogenase, organisms assimilating 0000-0-Sigel-ch07_R1_121004 Iron, Phytoplankton Growth, and Carbon Cycle 155 89 nitrate as a nitrogen source or fixing N2 from the atmosphere require much more 90 cellular iron to support growth than those utilizing reduced nitrogen species 91 [1,9–12]. 92 93 94 1.2. Cellular Uptake Mechanisms 95 By almost any measure, iron is vanishingly scarce in seawater, and much of the 96 iron that is present exists in forms not directly accessible to organisms (Sections 2 97 and 3). Marine phytoplankton are for the most part restricted to the uptake of dis- 98 solved Fe species although Fe can be solubilized from particles or colloids. 99 Marine phytoplankton have evolved several strategies for efficiently acquiring 100 iron and transporting it across the hydrophobic, semi-impermeable lipid bilayer 101 membrane surrounding most cells. The general mechanism involves the use of 102 cell-regulated membrane transport proteins (“transporters”), but prokaryotic 103 and eukaryotic cells have each developed distinct variations on this approach. 104 Prokaryotic microorganisms, including marine phototrophic and hetero- 105 trophic bacteria, are known to acquire Fe(III) by means of siderophore-based 106 transport systems. In these systems, cells under Fe stress synthesize and 107 release low-mass, high-affinity Fe(III)-chelating molecules (“siderophores”) 108 into the surrounding seawater, where they capture and solubilize otherwise una- 109 vailable Fe species (e.g., oxide minerals, particle-bound, organic complexes) 110 [13–16]. The Fe-siderophore chelates are then taken up via chelate-specific 111 transporters, which move the complex across the membrane and release Fe 112 inside the cell via reduction to Fe(II) or degradation of the siderophore itself. 113 Seawater surveys indicated that a large fraction of the bacteria were capable of 114 producing strong Fe-binding chelators (probably siderophores) [17–20]. Sidero- 115 phore transport systems, sometimes utilizing novel chelating agents, have been 116 described in many cyanobacteria [11,21,22] (Fig. 1). F1; Q1 117 In contrast to marine bacteria, there is little evidence for the production of 118 siderophores by eukaryotic marine phytoplankton [23,24]. Rather, eukaryotes 119 appear to utilize transporters that directly capture dissolved inorganic iron at 120 the cell membrane. Experiments with two diatoms and a coccolithophore 121 found that for these organisms Fe uptake depends on the concentration of 122 labile inorganic Fe and is independent of the concentration of total iron 123 [5,23,25]. Tracer experiments have shown that phytoplankton will make use of 124 iron bound to siderophores or other organic ligands only after photo- or biologi- 125 cally-mediated dissociation from the ligand [15]. It is likely that the transporters 126 involved in Fe uptake are transmembrane proteins, powered by ATP or cross- 127 membrane electrochemical gradients, and controlled by a DNA switch that 128 represses gene transcription and hence synthesis of transport proteins when 129 intracellular Fe concentrations become elevated [1,25,26]. 130 Certain classes of photosynthetic protozoan marine algae are able to 131 directly ingest cells or abiotic particles as supplementary sources of fixed 132 carbon and essential nutrients, including the bulk of the iron required to support photosynthesis and respiration [27]. These “mixotrophic” organisms 0000-0-Sigel-ch07_R1_121004 156 Street and Paytan 133 134 135 136 137 138 139 140 141 142 143 144 145 146 147 148 149 150 151 152 153 154 155 156 157 158 159 160 161 Figure 1 (A) Heme, one of several iron-bearing porphyrin compounds, occurring in 162 cytochromes and other metabolic proteins in marine microorganisms; (B) Alterobactin, 163 a siderophore produced by marine bacteria. Reproduced from Ref. [283] by courtesy of 164 the American Society of Limnology and Oceanography; (C) Chelation of Fe3þ by a hydro- 165 xamate siderophore. Adapted from Ref. [284] by courtesy of Marcel Dekker, Inc. 166 167 have been shown to be able to sustain moderate growth rates (35–70% of 168 maximum) using only ingested mineral colloids as Fe sources in seawater that 169 has been artificially depleted in dissolved iron [28]. 170 171 172 173 1.3. Chemical and Physical Limits on Uptake Rates 174 Iron uptake rates for most phytoplankton species appear to be limited by the rate 175 of cross-membrane transport, itself a function of ligand exchange kinetics and the 176 amount of space available on the outer cell membrane for transporter proteins 0000-0-Sigel-ch07_R1_121004 Iron, Phytoplankton Growth, and Carbon Cycle 157 177 [1,23,29]. This conclusion is born out by experiments with coastal diatoms and 178 dinoflagellates of a range of cell sizes (3.5–32 mm), which show that cell 179 surface- and volume-normalized Fe uptake rates, as well as specific growth 180 rates, flatten out with increasing dissolved Fe concentrations. Although part of 181 this effect may have resulted from Fe-hydroxide precipitation at high Fe concen- 182 trations, cell surface normalized Fe uptake was similar for all species, regardless 183 of size, consistent with the notion of uptake rates near their physical and chemical 184 limits [30]. This finding was replicated in a comparison of an oceanic diatom 185 (Thalassiosira oceanica) with a related coastal diatom (T.
Details
-
File Typepdf
-
Upload Time-
-
Content LanguagesEnglish
-
Upload UserAnonymous/Not logged-in
-
File Pages43 Page
-
File Size-