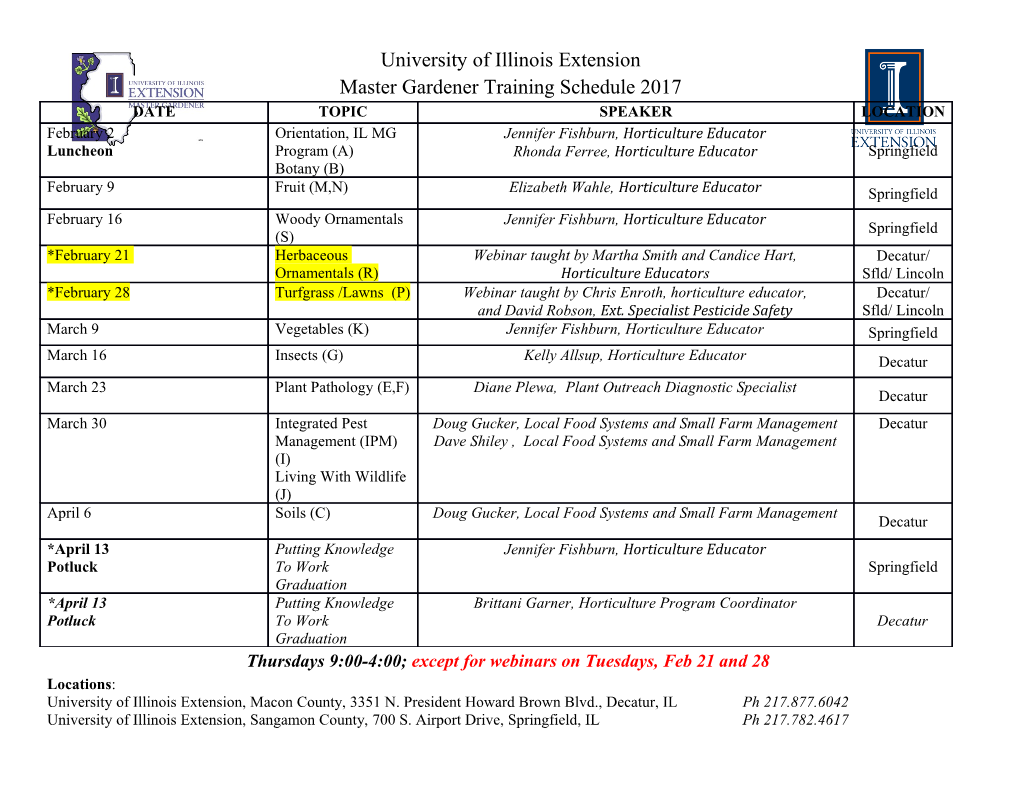
SPECIAL ISSUE On INTernAL WAveS Energy Release Through Internal Wave Breaking BY HANS VAN HAren AND LOUIS GOSTIAUX ABSTRACT. The sun inputs huge amounts of heat to the ocean, heat that would stay ABOVE | A large backward-breaking internal wave near the ocean’s surface if it were not mechanically mixed into the deep. Warm water is depicted in this time-depth image whose axes are 14 minutes horizontally and 50 m vertically. is less dense than cold water, so that heated surface waters “float” on top of the cold This wave was observed using 101 high-sampling- deep waters. Only active mechanical turbulent mixing can pump the heat downward. rate temperature sensors between 0.5 and 50 m above the bottom at 550 m depth near the top of Such mixing requires remarkably little energy, about one-thousandth of the heat Great Meteor Seamount, North Atlantic Ocean. stored, but it is crucial for ocean life and for nutrient and sediment transport. Several Temperature ranges from 12.3°C (blue) to 14.1°C mechanisms for ocean mixing have been studied in the past. The dominant mixing (red); contour intervals are every 0.1°C (black). mechanism seems to be breaking of internal waves above underwater topography. Here, we quantify the details of how internal waves transition to strong turbulent mixing by using high-sampling-rate temperature sensors. The sensors were moored above the sloping bottom of a large guyot (flat-topped submarine volcano) in the Canary Basin, North Atlantic Ocean. Over a tidal period, most mixing occurs in two periods of less than half an hour each. This “boundary mixing” dominates sediment resuspension and is 100 times more turbulent than open ocean mixing. Extrapolating, the mixing may be sufficiently effective to maintain the ocean’s density stratification. 124 Oceanography | Vol. 25, No. 2 INTRODUCTION they reach strong turbulence states above density contrast between different water Internal waves are ubiquitous around underwater topography (Thorpe, 1987a). layers generates weaker restoring forces. the globe in estuaries, seas, and oceans, The turbulence is visible as closed loops These internal waves have periods vary- causing their interiors to be in constant of a wide range of sizes. These loops can ing from one day, depending on Earth’s motion. They exist because these waters transport material and affect stratifica- rotation, down to several minutes, all have stable density stratification from tion. Quantification of such mixing pro- depending on stratification. surface to bottom. Ocean water density cesses thus requires detailed observations Low-frequency, long-period near- is mainly determined by temperature using a high sampling rate (once per sec- inertial internal wave motions have and salinity variations (Box 1). Vertical ond) and precise instrumentation. relatively small vertical wave lengths of density differences (“stratification”) create There are two dominant internal wave 10–100 m in the open ocean (1–10 m in a restoring force for wave motions in sources near the lowest internal wave fre- shallow seas). These small length scales the interior (“internal waves”). High- quency: “inertial” motions, which result cause the inertial currents to vary rapidly resolution temperature measurements from an adjustment to large-scale dis- in the vertical, creating large vertical cur- show the omnipresence of internal waves turbances such as fronts or atmospheric rent differences (termed shear or internal near beaches and at great depths, with storms in combination with Earth’s rota- friction). The resulting shear-induced similar features persisting at a wide vari- tion, and internal tides, which result from turbulent mixing occurs infrequently ety of scales, thus resembling a fractal the interaction of surface or “barotropic” in the open ocean, creating about one- world (Figure 1; note the 100–1,000 times tidal motions with topography. Whereas quarter to one-third (Gregg, 1989) of the different depth-time ranges and the fac- inertial motions can be observed domi- global, ocean-wide vertical turbulent dif- tor of 1,000 smaller temperature range in nantly near the ocean’s surface or in fusivity (a measure for vertical exchange –4 2 –1 panel d). Such features include overturn- closed seas like the Mediterranean with units of Kz = 10 m s ; Munk, ing of occasionally large 50 m high fronts (Figure 1d), internal tides are dominant 1966) needed to maintain deep-ocean (image on opposite page) followed by a in the open ocean (Figure 1a). Compared (heat) stratification. Hence, another trail of turbulent high-frequency internal to surface waves that are generated energy sink should exist, and much waves. The ocean interior’s 10–100 m through the much larger density dif- additional energy is lost near under- high weakly turbulent internal waves can ference between air and water, internal water topography such as seamounts thus mix and transport material when waves travel slowly because the lower and continental slopes. BOX 1 | TEMPERATURE AS A TRACER FOR DENSITY VARIATIONS The dynamics of vertically varying ocean motions depend on are the easiest to observe. Salinity is measured indirectly, using a variations in density. Though ocean density variations cannot be combination of temperature and conductivity sensors. Temperature measured directly, density is mainly (more than 99.99%) determined observations alone can be used at a particular known pressure by variations in temperature, salinity, and pressure. A vertically (depth) as a replacement (proxy) for density variations, provided stable water column is composed of warm, fresh water over cold, the density-temperature relationship is well known (tight). This salty water. Warmer water is less dense than colder water when relationship can be established by repeatedly lowering a shipborne salinity is 23 or more parts per thousand (ppt). An average ocean conductivity-temperature-pressure package at the site of interest. value is 35 ppt. Freshwater is less dense than salty water. Roughly 4°C If the vertical density variations are very small, about in temperature and 1 ppt in salinity each contribute to ±1 kg m–3 in 0.001 kg m–3 per 100 m, high-precision sensors can observe tem- density variations. Although the static pressure increases by 1 bar perature to increase with pressure (depth) while density remains (atmosphere) every 10 m vertically in the ocean, it is the small (1%) stable. The limit of this increase is the adiabatic lapse rate of about compressibility of water that affects density variations. 1.7 × 10–4 °C m–1, which is due to the compressibility of water. It can In terms of observational techniques, temperature and pressure be easily corrected for when pressure (depth) is known. Oceanography | June 2012 125 Because the interaction of horizontal However, it is unclear how energy trans- deforms the internal waves and makes motion with sloping underwater topog- fers from the internal wave source to them overturn. Another possible expla- raphy generates internal tides (part of its turbulence loss, but it is probably via nation is that high-frequency internal the low-frequency internal waves), it is small-scale internal waves. waves deform to linear or nonlinear conjectured that most mixing also occurs At the high-frequency end of the internal tides and then disintegrate into along the ocean’s sloping boundaries internal wave spectrum, short-period high-frequency solitary waves (Gerkema, rather than in its interior (Munk, 1966; waves near buoyancy frequency N are 1996; Farmer et al., 2011). Despite their Armi, 1978; Thorpe, 1987b; Garrett, the natural motions following stratifi- name, such high-frequency nonlinear 1990, 1991). The concentration of inter- cation disturbance (Groen, 1948). N 2 waves generally do not occur alone, but nal tidal energy may cause enhanced is proportional to the vertical density instead commonly occur in groups of wave breaking and mixing. Internal stratification and the acceleration of four to ten waves (Helfrich and Melville, waves constitute a partial (20%) sink gravity. These waves are close to turbu- 2006). When such solitary wave groups, for tidal energy, or about 0.6 TW glob- lence in frequency (D’Asaro and Lien, carried by the internal tidal wave, col- ally (Munk and Wunsch, 1998). If the 2000). One possible explanation for how lide with underwater topography, they boundary mixing described is efficient high-frequency internal waves transi- transform (Orr and Mignerey, 2003), NIOZ High Sampling Rate Thermistors enough, it should account for mean tion to turbulence is through interaction and the leading one sharpens into an energy loss (dissipation) of 1.5 mW m–2. with near-inertial or tidal shear, which upslope frontal bore (Vlasenko and Hutter, 2002; Klymak and Moum, 2003). a T (°C) b T (°C) Such a bore dominates sediment resus- −1400 10,000 s 6.7 100 s −0.8 18.2 6.6 pension (Hosegood et al., 2004; Bonnin 18.1 −1420 6.5 −1.0 et al., 2006), which resembles an atmo- 6.4 18.0 −1440 6.3 −1.2 ) ) 17.9 spheric dust storm. 6.2 z (m −1460 z (m −1.4 6.1 17.8 Here, we present detailed observa- −1480 6.0 −1.6 17.7 5.9 tions demonstrating that internal wave −1500 5.8 −1.8 17.6 5.7 motions above sloping topography are −2.0 17.5 −1520 212.8 213.0 213.2 213.4 213.6 180.334 180.335 180.336 t (yearday) t (yearday) indeed quite different from those in the ocean’s interior. High-resolution moored c T (°C) d ∆T (0.001°C) −500 1,000 s 14.0 −3980 100,000 s temperature sensors (Box 2) offer the −505 −3990 0.3 13.8 −510 −4000 0.2 best way to study the process of internal 13.6 −515 −4010 13.4 0.1 wave-turbulence dynamics.
Details
-
File Typepdf
-
Upload Time-
-
Content LanguagesEnglish
-
Upload UserAnonymous/Not logged-in
-
File Pages8 Page
-
File Size-