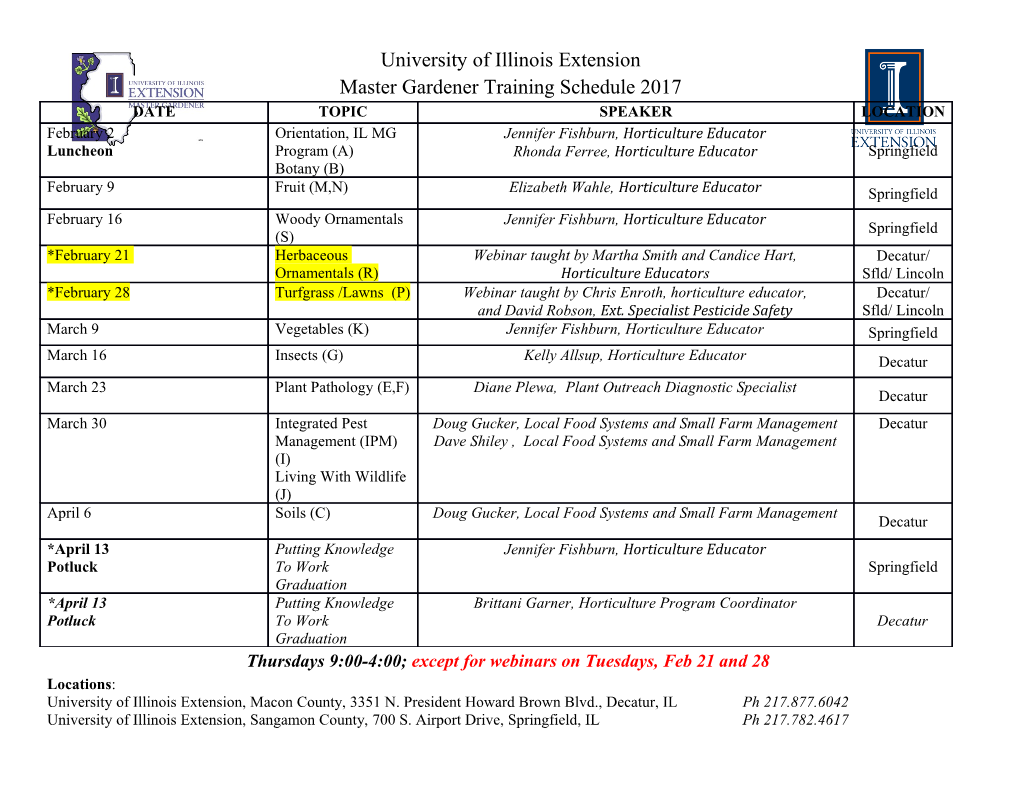
JUNE 2020 Mario Capecchi, Ph.D. NOT FOR PUBLIC DISTRIBUTION Michael Keel My name is Michael Keel from New Jersey. And it is my honor to introduce Dr. Mario Capecchi. Dr. Capecchi is the Distinguished Professor of Human Genetics at the University of Utah School of Medicine. He is best known for his groundbreaking work in gene targeting, in mass embryo derived stem cells. He was awarded the Nobel Prize in Physiology for Medicine, for his work in finding ways to manipulate the mammalian genome by changing mammals’ genes. His research interests include analysis of neural development in mammals, gene therapy, and production of murine models of human genetic diseases, from cancer to neuropsychiatric disorders. Dr. Capecchi, welcome to the Congress of Future Medical Leaders. Dr. Capecchi Thank you. First of all, welcome to the Medical Leaders’ Congress. It's a pleasure to be here. My name is Mario. I'm going to be talking about gene targeting. Next slide please. My laboratory has developed a technology called gene targeting that allows you to change any gene in any conceivable manner in a living creature, such as a mouse. Next slide. Why do we do gene targeting? Next slide. There are many reasons, but they boil down to two. One is basic research and the other is applied research. In basic research, you investigate the biology of an animal. For example, how do you make a limb or a heart or a brain? Those are basic research questions. The other is applied research. That is we can use gene targeting to generate mice with human diseases. Next slide please. There are over 5, 000 human diseases that cause, by single gene defects, that are caused by single gene defects. Next slide. Gene targeting allows us to generate mice, for example, with defined cancers, diabetes or neuropsychiatric disorders. Next slide. We can study disease in mice in much greater detail that is possible in humans for ethical reasons. Next slide please. The disease model can be first used to develop the how, the mechanism of disease starts from beginning to end when it shows the full pathology. Then once you understand the disease, you can use it as a platform to develop new therapies, the exact same mouse. Next slide please. In 1980, I submitted a grant to NIH proposing to develop the targeting intraembryonic cells. Next slide. The project was deemed impossible. Next slide. And the reason they gave for refusing the grant was that the newly introduced DNA that's being introduced into the living cell will never find the same sequence in the genome of a living cell. Since this is the very first step of gene targeting that said the project would not work. Next slide please. So I had two choices. One is to drop the project, or two is to continue the project using funds from another grant. Now the second possibility had enormous risk. Next slide, please. If I failed, I would be finished as a scientist. Imagine trying to get a grant after you've used their money for four years, and you have nothing to show for it. The next grant would be very difficult to ever receive. OFFICIAL TRANSCRIPT NATIONAL ACADEMY OF FUTURE PHYSICIANS AND MEDICAL SCIENTISTS © 2020 ALL RIGHTS RESERVED 1 JUNE 2020 However, I chose to go by this second route. Next slide, please. And the reason was the second option, if we were successful, the payoff would be enormous. And so we staked everything on that. Next slide, please. Four years later, we were successful. We could do gene targeting in a mirion cells. Next slide. This was fortunate because grants cover five years of work, but renewal occurs every four years. So you have to renew the grant every four years. And four years later, we had results and we could document that we could do gene targeting. Next slide, please. So I submitted a renewal to the same NIH section that refused the first grant, this time increasing the ante and proposing to do gene targeting not in mammalian cells, but in living mice. And their reply was Next slide, please. We're glad you didn't follow our advice. They have a sense of humor. And from then on, we could pursue it. Next slide, please. Now to understand the targeting, we need to appreciate the essence of DNA. Next slides. This shows you the structure. All of you are familiar with the DNA structure. This shows two DNA strands and they're bound together in a double helix. The red strand and a green strand. And what's holding them together is a ribose phosphate linkage, and then the basis, the information part, are the steps of that helix. Next slide, please. Now, the information content in DNA is the order of basis. And it's an alphabet four letters ATG and C rather than 26 letters. Next slide, please. And this shows you the essence. On the top, you have one strand of DNA, and then two base pairs, and that opposite strand. Okay, so we're looking at just two base pairs. And if there's a T on one strand, then on the other strand you automatically always have an A. Or if you have a C on one strand, on the other strand, you automatically have a G. And the two base pairs are held together by hydrogen bonds, which is shown in red. It's only this configuration of T and A or C and G, that allows the spacing to be exactly the same. And therefore you can construct a stable helix that's present in DNA. So this is everything that we ever do with DNA is dependent on this base pairing. That's the essence. Next slide, please. And this tells us right away how DNA functions to archive information. You simply have one double strand of DNA; they separate and each forms a template for making the opposite strand. And now you've duplicated with precision going from one molecule to two molecules, one mother cell to two daughter cells or one generation to the next generation. And this allows you to transfer the information from one generation to mother and father down to their siblings. Next slide please. Now, Gene tightening takes advantage of a process called homologous recombination. What is homologous recombination? Next slide. The major role of homologous recombination is to repair DNA damage. Next slide. However, at that time, when we started these experiments, that wasn't known. It only became apparent from our own experiments. And that's a separate story, which I don't have time to go into. Next slide, please. Now, in DNA, there are two major grooves, there are two grooves, a big one and a little one. The big one’s called the major groove, and the second one's called the minor groove. Next slide, please. Now, there's enough surface on, revealed on the major groove, to allow a molecular machine to identify which pair is present at any given position without having to separate the strands. So all you have to do is travel along the major groove, and you know exactly what sequence of DNA is through this machinery. Could I have the next slide please. And this homologous recombination return, it does something quite unexpected and magnificent. OFFICIAL TRANSCRIPT NATIONAL ACADEMY OF FUTURE PHYSICIANS AND MEDICAL SCIENTISTS © 2020 ALL RIGHTS RESERVED 2 JUNE 2020 When it finds two very long stretches of identical DNA sequence on homologous pairs of chromosomes. Remember, you always have two chromosomes in it for each for your information. It lines up the sequences, and then literally cuts and exchanges the strands with almost perfect precision. This process is shown in the next slide. Here, the top is a double helix, yellow, and then there's a blue one. They actually represent the exact same sequence, but I've covered one yellow, one blue, so you could distinguish them. So the sequence across that region is identical. Every base pair is identical to the other one, okay? Once they're lined up, and that's what the homologous combination does, then it literally cuts and rejoins them. And since we've made them different colors, you can see that they're now joined together with an opposite partner. But what's important is and in the process, it's done with perfection. So now the sequence is still the same from one end to the other on both strands. So it hasn't changed. It simply exchanged partners. Okay? And it's this machinery that we take advantage of, to generate, to do gene targeting. Now, the next slide, please. Okay, so this is what we're going to do. And then the next slide shows the actual mechanism. So here it is, it's very simple. On the top bar is your favorite gene. Okay. It represents, for example, an average gene would have 40, 000 letters. And in the middle of that, we've created this in a test tube. And in the middle of that we make a change and that's the asterisk, the red asterisk. Okay. And that change can be anything. A single base pair. 10 base pairs. A hundred. Thousands. And now we're working on a technology to make it many millions, so we can transfer enormous amounts of information to a single chosen spot on a chromosome. Okay, now, the asterisk is different. But on the sides of that asterisk is a sequence that is identical to what's present in the genome.
Details
-
File Typepdf
-
Upload Time-
-
Content LanguagesEnglish
-
Upload UserAnonymous/Not logged-in
-
File Pages6 Page
-
File Size-