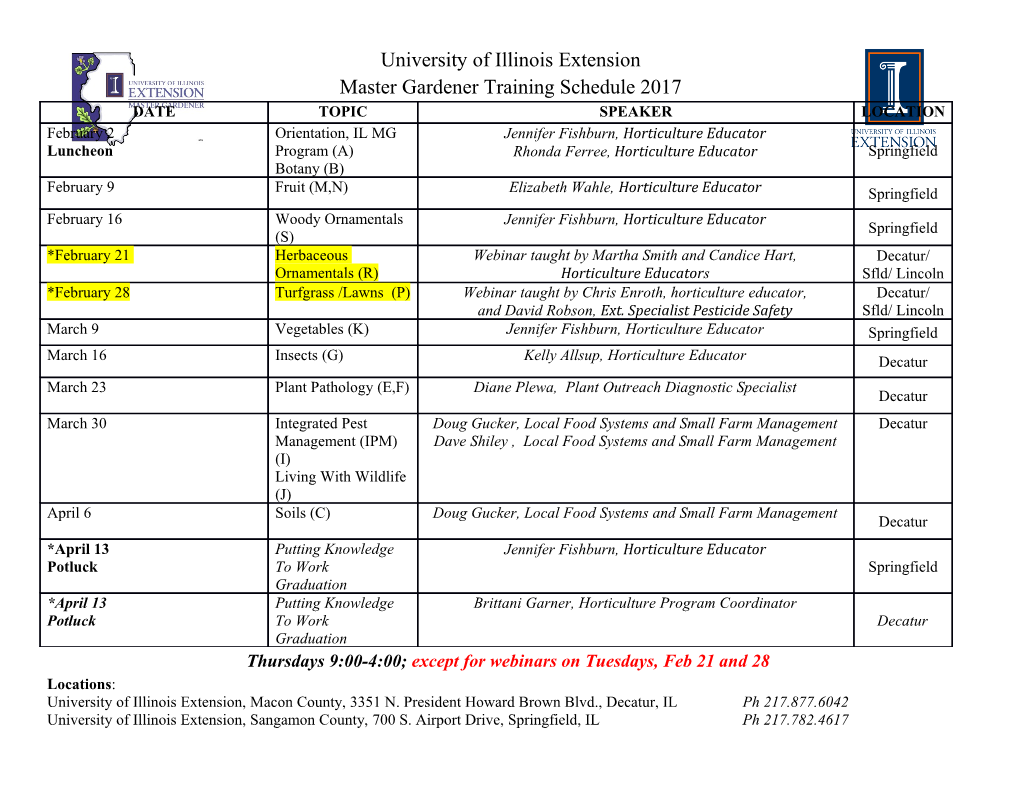
3.4 272 Voltage-Clamp and Patch-Clamp Techniques Hans Reiner Polder, Martin Weskamp, Klaus Linz, Rainer Meyer Introduction History of Membrane Potential and Current Recordings in Cardiac Tissue This chapter describes various methods of analysis of the electrical behaviour of ex- citable cells using microelectrodes. Since their introduction in the 1920’s, microelec- trodes have become the “workhorses” of electrophysiology, and a comprehensive number of publications exist about this topic. Here, we give a consolidated overview of the most important methods of investigation at single cell level, however, high throughput methods and automated recording procedures are not discussed. There is a reference list (Further Reading) at the end of this chapter, detailing some of the most important books published in recent decades. Since the nineteenth century, it has been well known that excitable cells are able to produce electrical signals. However, until the invention of the first glass microelec- trodes, by Ling and Gerard in 1949, the origin of these electrical signals could not be proven. For the first time, these glass micropipettes allowed the detection of the mem- brane potential of a cell, as their tips were small enough to penetrate the cell mem- brane without destroying the cells, and their high electrical resistance avoided shunting the membrane potential (see chapter Principles of Electrodes). These micro- electrodes were connected to the high resistance input of a voltage amplifier. The monitoring of the membrane potential, delivered the base of our actual understand- ing of electrical events inside the heart. In 1951, the first experiments on mammalian heart muscle tissue using these elec- trodes were published (Draper and Weidmann 1951). During their experiments, the negative membrane potential of around –80 mV and the cardiac action potential (AP), in its typical shape and with its real amplitude, could be recorded. Application of the voltage clamp technique (Cole 1949; Hodgkin et al. 1952), adapted to cardiac tissue (Deck et al. 1964), revealed the different current components of the cardiac action potential, which are described in chapter Membrane Currents During the Ac- tion Potential. A further leap in progress for cardiac physiology was gained by the de- velopment of techniques to isolate single living cardiac myocytes in the late 1970’s (Dow et al. 1981). At the same time, the patch clamp technique was invented (Hamill et al. 1981). Some small changes in the geometry of the electrodes, as well as the de- Voltage Clamp and Patch Clamp Techniques 273 3.4 velopment of new amplifiers (patch and single electrode clamp amplifiers), opened a completely new field as the recording of current through single channels became pos- sible. In addition, monitoring of membrane potential, as well as currents, was highly simplified. This allowed the description of new membrane currents and detailed analysis of their molecular basis, a process that still continues today. Description of Methods and Practical Approach Membrane Currents During the Action Potential The basic ionic currents during the AP were discovered by means of voltage clamp experiments on multicellular cardiac tissue preparations. Depolarisation, is caused by a voltage dependent sodium inward current, INa. Depolarisation, in turn, leads to a drop in K+ conductance, and the opening of voltage gated Ca2+ channels giving rise to an inward-calcium current, ICa. ICa lasts for less than twenty up to two hundred milli- seconds, depending on the species and thus AP- duration, e.g. murine AP-duration is around 40 ms (own unpublished observations) whereas the guinea-pig AP lasts for about 400 ms (Linz and Meyer, 1998a). The interplay of low K+ conductance and ICa keeps the membrane potential depolarised, and thus forms the plateau of the car- diac AP. During the plateau phase, the K+ conductance gradually re-increases, the ICa finally inactivates, and outward potassium currents repolarise the membrane. A detailed overview of cardiac excitation contraction coupling is presented in Bers (2002). As mentioned above, isolation of living cells and the invention of the patch clamp technique simplified experiments on cardiac myocytes, thus our knowledge of cellu- lar electrical events in the heart has blown up in the last twenty years. Therefore, some more details have to be added to the concept mentioned above. The role of the 2+ depolarising INa remains unchanged. As a family of Ca currents in many different cells has been discovered, it has become clear that the most important current in car- diac myocytes is the L-type current, ICa,L (see chapter 3.5). In addition, a so-called high voltage activated Ca2+ current, the T-type current, is expressed in cardiac myocytes. The concept of K+ currents has become relatively complicated in recent years. Differ- ences in AP shape between species and cardiac tissues like pacemaker cells, cells of conductive tissue, atrial and ventricular myocytes, depend mainly on the expression of different types of K+ channels. As the diversity of K+ currents is not the topic of this report, only a few aspects will be mentioned here (for a more detailed review see chapter 3.5). In many species a transient outward current Ito is activated very rapidly. This current induces an initial repolarisation after the peak of the AP. The ampli- tude of Ito determines the potential at which the plateau starts, and thereby Ito also determines duration and shape of the AP. The final repolarisation is brought about + by two slowly activating K current components, IKr and IKs. At potentials negative + of –50 mV, IK1 becomes the dominating K current, determining the resting po- tential. 3 274 Electrophysiological Techniques Principles of Electrodes Since the invention of glass microelectrodes, different forms have been developed, however, all follow the same principles as described in this chapter. “Microelectrodes” are micropipettes made of glass, filled with electrolytic solutions, with an electric connection to the recording amplifier. The microelectrode is in direct contact with the cell interior, either by penetration of the cell membrane (“sharp microelectrode re- cordings”), or by rupturing the membrane inside a suction electrode sealed to the sur- face of the cell (whole cell patch configuration). In case of a “perforated patch”, some pore forming agents are used in the pipette solution to give access to the cell interior (see chapter Perforated Patch). Microelectrodes consist of the stem, the shoulder, the taper and the tip (Fig. 1). The stem with the constant diameter is the longest part of the microelectrode, followed by the shoulder and then the taper that has a continu- ously decreasing diameter. The taper ends in a fine tip. Depending on the diameter of the tip, microelectrodes are termed “sharp” (0.5 µm or less) or “patch” electrodes (typically 1 to 3 µm, fire polished). Microelectrodes are filled with electrolyte solution (see below). Normally, an Ag-AgCl wire is immersed into the electrolyte, connecting the electrode to the recording amplifier. Thus, the microelectrode converts ionic cur- rent in solution, into an electron current in wires, according to the following revers- ible reaction: Cl– + Ag AgCl + e– In-Vitro Techniques Figure 1a–d Microelectrode. a, b Scanning electron micro- scopic images of the tip of a sharp microelec- trode. Scale a: 10 µm; Scale b: 1 µm. c, d Light microscopic images of a patch clamp electrode. c Displays in low magnification the taper and the tip, scale 500 µm. d Tip of same electrode in higher magnification, scale 10 µm. Filled with 135 K+, Na+, 135 Cl– 10 HEPES, 0.5 EGTA, all in mM, the electrode had a resistance of 4 MΩ Voltage Clamp and Patch Clamp Techniques 275 3.4 Figure 2 Equivalent circuit microelec- trode (ME). Terminology and equivalent circuit of a micro- electrode. CEL electrode capacity, REL electrode resistance, TP tip potential Chloriding of silver wires can easily be done by electrolysis. Many available microelec- trode holders have built-in Ag-AgCl pellets, which are a good but more expensive al- ternative to chlorided silver wires. In terms of electrochemistry, only this part is an “electrode”. In electrophysiology, the terms “microelectrode”, “patch” or “suction elec- trode”, are used for the glass micropipette and the electrochemical electrode. In most cases, the reference (“ground signal”) for the measurement is the bath surrounding the cell. The electrical connection is also made via chlorided silver wire or an Ag-AgCl pellet. This reduces a part of the occurring offset potentials. Offset potential compensation is a very important procedure, and can be done prior to an experiment if all offsets are constant. In case of solution changes, offset potentials will also change and therefore correction procedures may be necessary (Neher 1995). Electrical Properties From an electrical point of view, microelectrodes are RC-elements (Fig. 2) with a bat- tery. The resistance (R), capacitance (C), and electrode potential are mostly non-lin- ear and spread along the thin part of the microelectrode. The most unpleasant non-linearity is often called “rectification”, this is because the microelectrode resis- tance varies depending on the direction of current flow. Beside mechanical sensitiv- ity, these factors are serious limitations, which require sophisticated recording electronics. A simplified equivalent circuit diagram shows a resistor in parallel to a capacitor and in series to a source of voltage, the tip potential. Microelectrodes are selected carefully to have linear electrical properties in the range of operation. There- fore, the non-linear electrical properties (rectification of electrodes) are usually of minor practical importance and are omitted. The microelectrode resistance Rel is basically dependent on the glass species, the diameter and length of the tip, the concentration of the electrolyte inside the micro- electrode, and the concentration of the solution into which the microelectrode is im- mersed. The lower the concentration of the filling solution and the finer the diameter 3 276 Electrophysiological Techniques of the tip, generally, the higher the microelectrode resistance.
Details
-
File Typepdf
-
Upload Time-
-
Content LanguagesEnglish
-
Upload UserAnonymous/Not logged-in
-
File Pages52 Page
-
File Size-