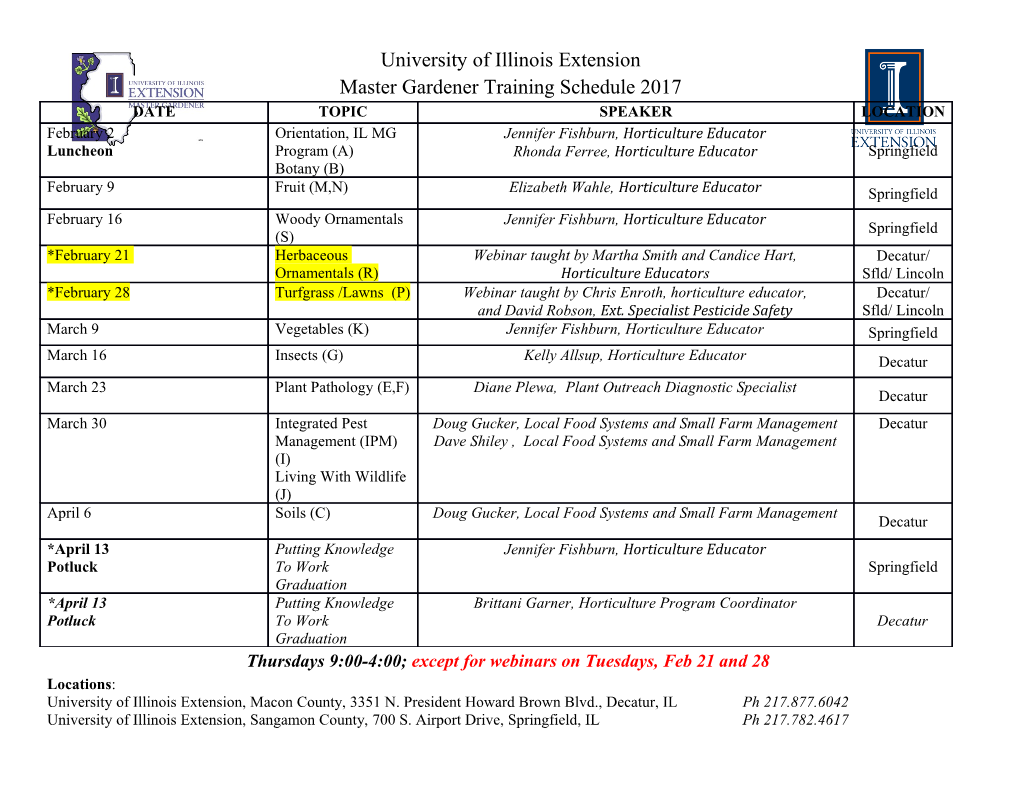
Lectures on The Riemann Zeta–Function By K. Chandrasekharan Tata Institute of Fundamental Research, Bombay 1953 Lectures on the Riemann Zeta-Function By K. Chandrasekharan Tata Institute of Fundamental Research 1953 The aim of these lectures is to provide an intorduc- tion to the theory of the Riemann Zeta-function for stu- dents who might later want to do research on the subject. The Prime Number Theorem, Hardy’s theorem on the Zeros of ζ(s), and Hamburger’s theorem are the princi- pal results proved here. The exposition is self-contained, and required a preliminary knowledge of only the ele- ments of function theory. Contents 1 The Maximum Principle 1 2 The Phragmen-Lindelof principle 9 3 Schwarz’s Lemma 17 4 Entire Functions 25 5 Entire Functions (Contd.) 35 6 The Gamma Function 45 1 Elementary Properties . 45 2 Analytic continuation of Γ(z)............... 49 3 TheProductFormula ................... 51 7 The Gamma Function: Contd 55 4 The Bohr-Mollerup-Artin Theorem . 55 5 Gauss’s Multiplication Formula . 58 6 Stirling’s Formula . 59 8 The Zeta Function of Riemann 63 1 Elementary Properties of ζ(s)............... 63 9 The Zeta Function of Riemann (Contd.) 69 2 Elementary theory of Dirichlet Series . 69 v vi Contents 10 The Zeta Function of Riemann (Contd) 75 2 (Contd). Elementary theory of Dirichlet series . 75 11 The Zeta Function of Riemann (Contd) 87 3 Analytic continuation of ζ(s). First method . 87 4 Functional Equation (First method) . 90 5 Functional Equation (Second Method) . 92 12 The Zeta Function of Riemann (Contd) 97 6 Some estimates for ζ(s).................. 97 7 Functional Equation (Third Method) . 99 13 The Zeta Function of Riemann (Contd) 105 8 The zeros of ζ(s) .....................105 14 The Zeta Function of Riemann (Contd) 113 9 Riemann-Von Magoldt Formula . 113 15 The Zeta Function of Riemann (Contd) 121 10 Hardy’sTheorem . .121 16 The Zeta Function of Riemann (Contd) 127 17 The Zeta Function of Riemann (Contd) 135 12 The Prime Number Theorem . 135 13 Prime Number Theorem and the zeros of ζ(s) ......145 14 Prime Number Theorem and the magnitude of pn . 146 Lecture 1 The Maximum Principle Theorem 1. If D is a domain bounded by a contour C for which Cauchy’s 1 theorem is valid, and f is continuous on C regular in D, then “ f M | | ≤ on C” implies “ f M in D”, and if f = M in D, then f is a constant. | |≤ | | Proof. (a) Let zo D, n a positive integer. Then ∈ n n 1 f (z) dz f (zo) = { } | | 2πi z zo C − n lc M · , ≤ 2πδ where lc = length of C, δ = distance of zo from C. As n →∞ f (z) M. | |≤ (b) If f (zo) = M, then f is a constant. For, applying Cauchy’s inte- | | d gral formula to f (z) n , we get dz { } 1 f ndz n f (z ) n 1 f (z ) = o − ′ o 2 | { } · | 2πi (z zo) C − l Mn C , ≤ 2πδ2 1 2 1. The Maximum Principle so that lc M 1 f ′(zo) 0, as n | |≤ 2πδ2 · n → →∞ Hence f ′(zo) = 0. | | 2 (c) If f (zo) = M, and f (zo) = 0, then f (zo) = 0, for | | | ′ | ′′ 2 d n n 2 2 n 1 f (z) = n(n 1) f (z) − f ′(z) + n f (z) − f ′′(z). dz2 { } − { } { } { } At zo we have 2 d n n 1 f (z) = = n f − (Z0) f ′′(z0), dz2 { } z zo so that 2! f (z) ndz nMn 1 f (z ) = − ′′ 0 { } 3 | | 2πi (z zo) C − 2!l c Mn, ≤ 2πδ3 and letting n , we see that f (zo) = 0. By a similar reasoning →∞ ′′ we prove that all derivatives of f vanish at z0 (an arbitrary point of D). Thus f is a constant. Remark. The above proof is due to Landau [12, p.105]. We shall now show that the restrictions on the nature of the boundary C postulated in the above theorem cab be dispensed with. Theorem 2. If f is regular in a domain D and is not a constant, and M = max f , then z D | | ∈ f (zo) < M, zo D. | | ∈ For the proof of this theorem we need a 1. The Maximum Principle 3 Lemma. If f is regular in z z0 r, r > 0, then | − |≤ f (z0) Mr, | |≤ 3 where Mr = max f (z) , and f (zo) = Mr only if f (z) is constant for z zo =r | | | | | − | z zo = r. | − | Proof. On using Cauchy’s integral formula, we get 1 f (z) f (zo) = dz 2πi z z0 z zo =n − | − | 2π 1 = f (z + reiθ)dθ. (1) 2π o 0 Hence f (zo) Mr. | |≤ Further, if there is a point ζ (such that) ζ zo = r, and f (ζ) < | − | | | Mr, then by continuity, there exists a neighbourhood of ζ, on the circle z zo = r, in which f (z) Mr ε, ε > 0 and we should have | − | | | ≤ − f (zo) < Mr; so that “ f (zo) = Mr” implies “ f (z) = Mr everywhere on | | | | iθ | | z zo = r”. That is f (zo + re ) = f (zo) for 0 θ 2π, or | − | | | | | ≤ ≤ iθ iϕ f (zo + re ) = f (zo)e , 0 ϕ 2π ≤ ≤ On substituting this in (1), we get 2π 1 1 = cos ϕdθ 2π 0 Since ϕ is a continuous function of θ and cos ϕ 1, we get cos ϕ 1 for ≤ · all θ, i.e. ϕ = 0, hence f (s) is a constant. Remarks. The Lemma proves the maximum principle in the case of a 4 circular domain. An alternative proof of the lemma is given below [7, Bd 1, p.117]. 4 1. The Maximum Principle iθ Aliter. If f (z0 + re ) = φ(θ) (complex valued) then 2π 1 f (z ) = φ(θ)dθ 0 2π 0 Now, if a and b are two complex numbers, a M, b M and | | ≤ | | ≤ a , b, then a + b < 2M. Hence if φ(θ) is not constant for 0 θ < 2π, | | ≤ then there exist two points θ1, θ2 such that φ(θ1) + φ(θ2) = 2Mr 2ε, say, where ε> 0 | | − On the other hand, by regularity, φ(θ1 + t) φ(θ1) < ε/2, for 0 < t <δ | − | φ(θ2 + t) φ(θ2) < ε/2, for 0 < t <δ | − | Hence φ(θ1 + t) + φ(θ2 + t) < 2Mr ε, for 0 < t < δ. | | − Therefore 2π θ1 θ1+δ θ2 θ2+δ 2π φ(θ)dθ = + + + + 0 0 θ1 θ1+δ θ2 θ2+δ θ1 θ2 2π δ = + + φ(θ)dθ + [φ(θ + t) + φ(θ + t)] dt 1 2 0 θ+δ θ+δ 0 1 2 Hence 2π φ(θ) dθ Mr(2π 2δ) + (2Mr ε)δ = 2πMr εδ | |≤ − − − 0 2π 1 φ(θ)dθ < Mr |2π | 0 1. The Maximum Principle 5 5 Proof of Theorem 2. Let zo D. Consider the set G1 of points z such ∈ that f (z) , f (zo). This set is not empty, since f is non-constant. It is a proper subset of D, since zo D, zo < G1. It is open, because f is ∈ continuous in D. Now D contains at least one boundary point of G1. For if it did not, G D would be open too, and D = (G1 G )D would be 1′ ∩ ∪ 1′ disconnected. Let z1 be the boundary point of G1 such that z1 D. Then ∈ z1 < G1, since G1 is open. Therefore f (z1) = f (zo). Since z1 BdG1 ∈ and z1 D, we can choose a point z2 G1 such that the neighbourhood ∈ ∈ of z1 defined by z z1 z2 z1 | − | ≤ | − | lies entirely in D. However, f (z2) , f (z1), since f (z2) , f (zo), and f (zo) = f (z1). Therefore f (z) is not constant on z z1 = r, (see | − | (1) on page 3) for, if it were, then f (z2) = f (z1). Hence if M′ = max f (z) , then z z1 = z2 z1 | | | − | | − | f (z1) < M′ M = max f (z) | | ≤ z D | | ∈ i.e. f (zo) < M | | Remarks. The above proof of Theorem 2 [7, Bd I, p.134] does not make use of the principle of analytic continuation which will of course provide an immediate alternative proof once the Lemma is established. Theorem 3. If f is regular in a bounded domain D, and continuous in D,¯ then f attains its maximum at some point in Bd D, unless f is a constant. Since D is bounded, D¯ is also bounded. And a continuous function on a compact set attains its maximum, and by Theorem 2 this maximum 6 cannot be attained at an interior point of D. Note that the continuity of f is used. | | Theorem 4. If f is regular in a bounded domain D, is non-constant, and if for every sequence zn ,zn D, which converges to a point ξ Bd D, { } ∈ ∈ we have lim sup f (zn) M, n | |≤ →∞ then f (z) < M for all z D. [17, p.111] | | ∈ 6 1. The Maximum Principle Proof. D is an Fσ, for define sets Cn by the property: Cn consists of all z such that z n and such that there exists an open circular neigh- | | ≤ bourhood of radius 1/n with centre z, which is properly contained in D. Then Cn Cn+1, n = 1, 2,...; and Cn is compact. ⊂ Define max f (z) mn. Z Cn | |≡ ∈ By Theorem 2, there exists a zn Bd Cn such that f (zn) = mn. The ∈ | | sequence mn is monotone increasing, by the previous results; and the { } sequence zn is bounded, so that a subsequence zn converges to a { } { p } limit ξ Bd D. Hence ∈ lim f (zn ) M | p |≤ i.e.
Details
-
File Typepdf
-
Upload Time-
-
Content LanguagesEnglish
-
Upload UserAnonymous/Not logged-in
-
File Pages154 Page
-
File Size-