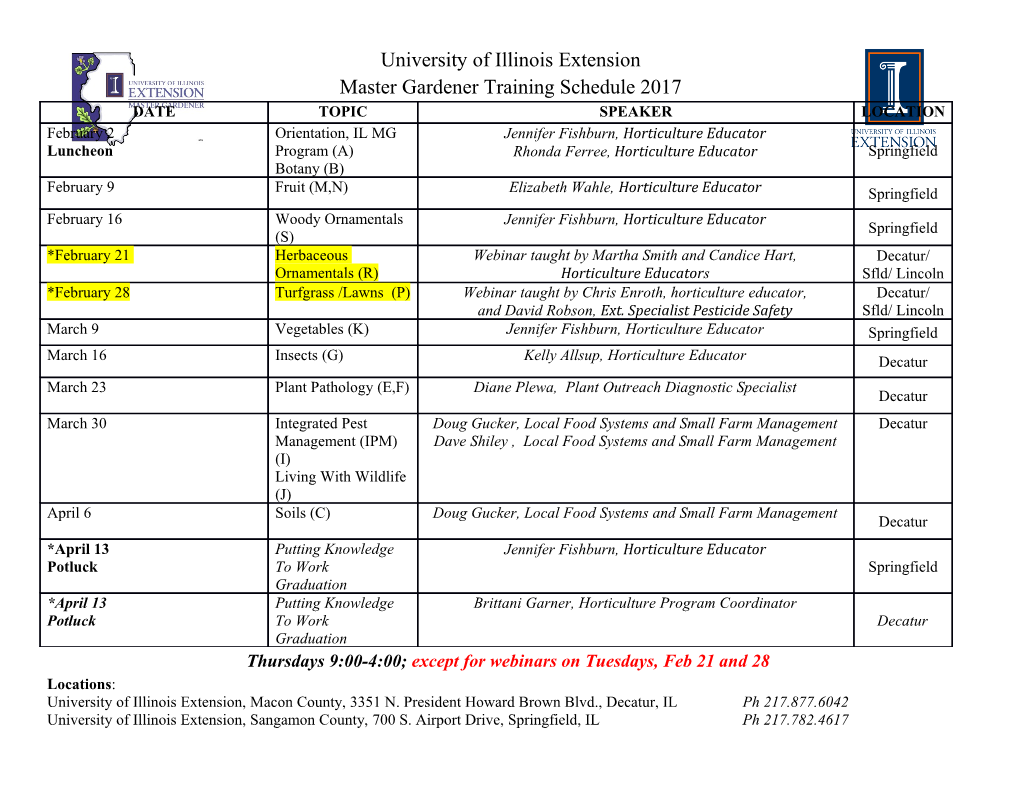
Dynamic X-ray diffraction observation of shocked solid iron up to 170 GPa Adrien Denoeuda,b,1, Norimasa Ozakic,d, Alessandra Benuzzi-Mounaixa,b, Hiroyuki Uranishic, Yoshihiko Kondoc, Ryosuke Kodamac,d, Erik Brambrinka,b, Alessandra Ravasioa,b, Maimouna Bocouma,b, Jean-Michel Boudennea,b, Marion Harmande, François Guyote, Stephane Mazevetf, David Rileyg, Mikako Makitag, Takayoshi Sanoh, Youichi Sakawah, Yuichi Inubushii,j, Gianluca Gregorik, Michel Koeniga,b,l, and Guillaume Morarde aLaboratoire d’Utilisation de Lasers Intenses - CNRS, Ecole Polytechnique, Commissariat à l’Energie Atomique et aux Energies Alternatives, Université Paris- Saclay, F-91128 Palaiseau Cedex, France; bSorbonne Universités, Université Pierre et Marie Curie Paris 6, CNRS, Laboratoire d’Utilisation des Lasers Intenses, place Jussieu, 75252 Paris Cedex 05, France; cGraduate School of Engineering, Osaka University, Osaka 5650871, Japan; dPhoton Pioneers Center, Osaka University, Osaka 5650871, Japan; eInstitut de Minéralogie, de Physique des Matériaux et de Cosmochimie, Sorbonne Universités – Université Pierre et Marie Curie, CNRS, Muséum National d’Histoire Naturelle, Institut de Recherche pour le Développement, 75005 Paris, France; fLaboratoire Univers et Théories, Observatoire de Paris, CNRS, Université Paris Diderot, 92195 Meudon, France; gCentre for Plasma Physics, School of Maths & Physics, Queens University Belfast, Belfast BT7 1NN, Northern Ireland; hInstitute of Laser Engineering, Osaka University, Osaka 5650871, Japan; iRIKEN SPring-8 Center, Hyogo 679-5148, Japan; jJapan Synchrotron Radiation Research Institute, Hyogo 679-5198, Japan; kDepartment of Physics, University of Oxford, Oxford OX1 3PU, United Kingdom; and lInstitute for Academic Initiatives, Osaka University, Osaka 565-0871, Japan Edited by Vladimir E. Fortov, Russian Academy of Sciences, Moscow, and approved May 31, 2016 (received for review June 22, 2015) Investigation of the iron phase diagram under high pressure and difference in melting temperatures between different studies (1, 2, temperature is crucial for the determination of the composition 4, 26, 27). This ongoing controversy could be related to various of the cores of rocky planets and for better understanding the problems of gradients and inhomogeneities in the studied material generation of planetary magnetic fields. Here we present X-ray as well as of chemical reaction and thermal diffusion with the di- diffraction results from laser-driven shock-compressed single-crystal amonds. Moreover, static compression methods do not allow, so and polycrystalline iron, indicating the presence of solid hexagonal far, reaching in association with precise measurements pressures close-packed iron up to pressure of at least 170 GPa along the and temperatures over 300 GPa and 5,000 K, respectively. Indeed, principal Hugoniot, corresponding to a temperature of 4,150 K. This only a few data are actually available over 200 GPa and high EARTH, ATMOSPHERIC, is confirmed by the agreement between the pressure obtained from temperature on pure iron (11, 12). For all of these reasons, it thus AND PLANETARY SCIENCES the measurement of the iron volume in the sample and the inferred becomes necessary to perform dynamic compression to reach higher shock strength from velocimetry deductions. Results presented in temperature/pressure conditions of relevance for planetology. This this study are of the first importance regarding pure Fe phase can be done with high-power lasers by using shock or quasi-isen- diagram probed under dynamic compression and can be applied to tropic compression techniques (28, 29). Current discrepancies ob- study conditions that are relevant to Earth and super-Earth cores. served between shock and static studies of iron over 100 GPa have been attributed to nonequilibrium processes (30), casting doubts on X-ray diffraction | iron phase diagram | shock-compressed iron | the possibility of properly probing phase diagrams of geophysically Earth core | dynamic compression relevant materials using dynamic compression. Different features, such as overheating or presence of a new crystalline structure in iron ron is the main constituent of Earth and telluric planetary cores. just below the melting curve, have been described in shock experi- ITherefore, because of this geophysical interest, its phase diagram ments in contradiction with static experiments. Here we aim at under high pressure and temperature has been extensively studied by providing new data on this question by probing shock-compressed static (1–4) or dynamic (5–9) compression techniques. In particular, the determination of its melting curve at the Earth’sinner−outer core Significance boundary constrains the thermodynamics that governs Earth’s heat budget and dynamics such as the heat flux from the core to the mantle, Iron is the main constituent of the core of rocky planets; therefore, the power available for the geodynamo, and the planetary cooling rate understanding its phase diagram under extreme conditions is (10). Besides, the knowledge of the exact crystalline structure of iron at fundamental to model the planets’ evolution. Using dynamic appropriate pressures and temperatures is a key parameter to explain compression by laser-driven shocks, pressure and temperature seismological observations. On this point, contradictory results have conditions close to what is found in these cores can be reached. been published concerning the crystallographic structure of iron under However, it remains unclear whether phase boundaries determined Earth’s inner core conditions (360 GPa and ∼6,000 K). Recent static at nanosecond timescales agree with static compression. Here we compression on iron (11) and iron alloys (12) has highlighted the observed the presence of solid hexagonal close-packed iron at 170 stability of hexagonal-close-packed (hcp) structure under extreme GPaand4,150K,inapartoftheironphasediagram,whereeithera conditions, whereas previous studies investigated the potential stabili- different solid structure or liquid iron has been proposed. This X-ray zation of double hcp (dhcp) or body-centered cubic (bcc) structures diffraction experiment confirms that laser compression is suitable under high pressure and temperature (13–17). However, having an for studying iron at conditions of deep planetary interiors difficult hcp structure under Earth’s inner core conditions is mandatory to to achieve with static compression techniques. explain its anisotropic structure observed by seismology (18, 19). Iron and its alloys have been widely studied by static compression Author contributions: A.D., N.O., A.B.-M., H.U., Y.K., M.K., and G.M. designed research; A.D., N.O., A.B.-M., H.U., Y.K., E.B., A.R., M.B., M.H., M.M., T.S., Y.S., Y.I., and G.M. in associating large volume cells or diamond anvils cells (DAC) with performed research; N.O., A.B.-M., R.K., E.B., J.-M.B., F.G., S.M., D.R., G.G., M.K., and a probing synchrotron radiation source (20, 21). In addition to the G.M. contributed new reagents/analytic tools; A.D., H.U., and Y.K. analyzed data; and study of the solid−solid phase transitions, like bcc−face-centered A.D., N.O., and G.M. wrote the paper. cubic (fcc) in temperature and bcc−hcpinpressure,andtothe The authors declare no conflict of interest. measurement of some properties of these phases (11, 22, 23), X-ray This article is a PNAS Direct Submission. diffraction also allows the study of solid−liquid transitions (24) and 1To whom correspondence should be addressed. Email: [email protected]. measurement of liquid density (25). Static compression measure- This article contains supporting information online at www.pnas.org/lookup/suppl/doi:10. ments of the melting curve of iron have led to over 1,000 K 1073/pnas.1512127113/-/DCSupplemental. www.pnas.org/cgi/doi/10.1073/pnas.1512127113 PNAS Early Edition | 1of5 Downloaded by guest on September 25, 2021 Fig. 1. (A) Experimental setup and target configuration at GEKKO XII target chamber. The incident angles of the shock drive beams (SD) and X-ray source beams (XS) were 30° from the normal to the target. The angular-resolved spectrometer was made by a defocused cylindrical Von Hamos geometry. The HOPG crystal and the image plate were surrounded by a 2-mm stainless steel box. (B) Magnified top view of the target. (C) Magnified bottom view of the target. X-rays from the Fe source target were collimated by the input and output pinholes of 200 μm diameter. Because the accuracy of the target fabrication was better than 10 μm, the X-ray beam irradiated appropriately the central region of shock wave driven by the SD beams (1-mm spot diameter). Moreover, to produce monochromatic laser plasma X-rays, we inserted a 125-μm-thick polyamide foil and a 10-μm-thick iron foil into the 1-mm gap between the pinholes. iron at the nanosecond and picosecond timescales using X-ray dif- of separating the different spectral components can significantly fraction (XRD), thus providing a direct measurement of its reduce the uncertainties in identifying the elastic signal contribution structure. and thus increase accuracy in structure determination. Structural XRD studies of iron at nanosecond or better time res- Two diffraction experiments were performed using different time olution have been, so far, focused on the low-pressure bcc−hcp durations for the X-ray probe. The nanosecond resolution XRD transition and on measuring the axial ratio (c/a) of the hcp phase at experiment was performed on the GEKKO XII laser facility at In- low pressure
Details
-
File Typepdf
-
Upload Time-
-
Content LanguagesEnglish
-
Upload UserAnonymous/Not logged-in
-
File Pages5 Page
-
File Size-