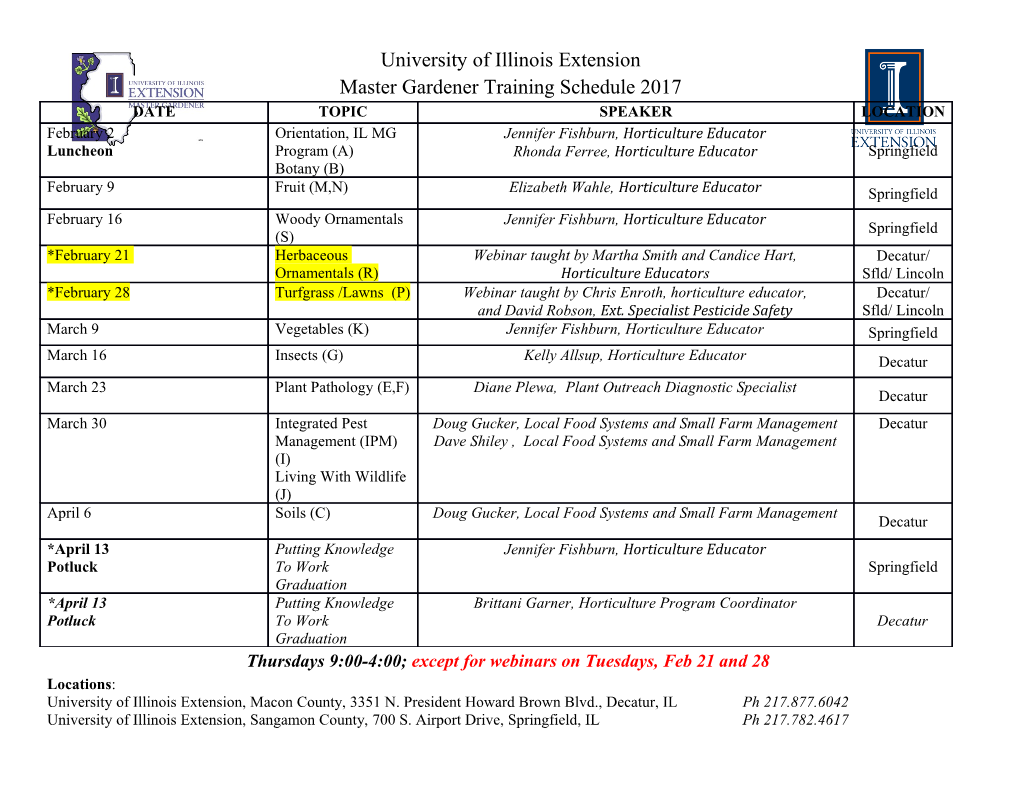
Single Photon Interferometry and Quantum Astrophysics by Genady Pilyavsky A Dissertation Presented in Partial Fulfillment of the Requirements for the Degree Doctor of Philosophy Approved November 2018 by the Graduate Supervisory Committee: Philip Mauskopf, Chair Christopher Groppi Nathaniel Butler Judd Bowman Paul Scowen ARIZONA STATE UNIVERSITY December 2018 ABSTRACT This thesis contains an overview, as well as the history of optical interferometers. A new approach to interferometric measurements of stars is proposed and explored. Modern updates to the classic techniques are described along with some theoreti- cal derivations showing why the method of single photon counting shows significant promise relative to the currently used amplitude interferometry. Description of a modular intensity interferometer system using commercially avail- able single-photon detectors is given. Calculations on the sensitivity and uv-plane coverage using these modules mounted on existing telescopes on Kitt Peak, Arizona is presented. Determining fundamental stellar properties is essential for testing models of stellar evolution as well as for deriving physical properties of transiting exoplanets. The proposed method shows great promise in measuring the angular size of stars. Simulations indicate that it is possible to measure stellar diameters of bright stars with AB magnitude ≤6 with a precision of ≥5% in a single night of observation. Additionally, a description is given of a custom time-to-digital converter designed to time tag individual photons from multiple single-photon detectors with high count rate, continuous data logging, and low systematics. The instrument utilizes a tapped- delay line approach on an FPGA chip which allows for sub-clock resolution of <100 ps. The TDC is implemented on a Re-configurable Open Architecture Computing Hardware Revision 2 (ROACH2) board which allows for continuous data streaming and time tagging of up to 20 million events per second. The functioning proto- type is currently set-up to work with up to ten independent channels. Laboratory characterization of the system, including RF, pick up and mitigation, as well as mea- surement of in-lab photon correlations from an incoherent light source (artificial star), are presented. Additional improvements to the TDC will also be discussed, such as improving the data transfer rate by a factor of 10 via an SDP+ Mezzanine card and i PCIe 2SFP+ 10 Gb card, as well as scaling to 64 independent channels. Furthermore, a modified nulling interferometer with image inversion is proposed, for direct imaging of exoplanets below the canonical Rayleigh resolution limit. Image inversion interferometry relies on splitting incoming radiation from a source, either spatially rotating or reflecting the electric field from one arm of the interferometer before recombining the signals and detecting the resulting images in the two output ports with an array of high-speed single-photon detectors. Sources of incoming radia- tion that have cylindrical symmetry and are centered on the rotation axis will cancel in one of the output ports and add in the other output port. The ability to suppress light from a host star, as well as the ability to resolve past the Rayleigh limit, enables sensitive detection of exoplanets from a stable environment without the need for a coronagraph. The expected number of photons and the corresponding variance in the measurement for different initial contrast ratios are shown, with some first-order theoretical instrumental errors. Lastly, preliminary results from a sizeable photometric survey are presented. This survey is used to derive bolometric flux alongside from angular size measurements and the effective stellar temperatures. ii Acknowledgment I like to think of this section as an extended author list, as multiple people through- out my life have contributed in one way or another, to the completion of this thesis. For the sake of organization, and in order to minimize the unavoidable omission of individuals, I will attempt to go in the quasi-chronological order. None of this would have been possible without the love, support and encourage- ment from my family. I am grateful for bedtime stories of aliens and stars that first sparked my interest in the cosmos. Your persistent emphasis on education had led me on this path. By design or by accident, you have shaped me into the person that I am, a perfect being and one of the most humble, modest individuals on this Earth. Post-undergraduate work and mentorship under Stephen Kane and Gerard T. van Belle convinced me to not only pursue a Ph.D. but that I may succeed. Thank you, Natalie Hinkel, for becoming my big sister, encouraging me to attend Arizona State University, and guiding me along the way. Caleb Wheeler, you have tough me plenty of valuable lessons, including the importance of putting things back, and putting things in boxes. Thanks go out to my committee members, Judd Bowman, Nathaniel Butler, Paul Scowen, Christopher Groppi, and my advisor Philip Mauskopf. Judd, thank you for putting up with me rambling incoherently about ad-hoc ideas and Nat for proposing though provoking questions that would change my perception of the physics behind this thesis. Paul, on numerous occasions, your accessibility has steered me in the right direction and allowed me to carry forth with my project. Chirs, you wisdom assisted me in learning how research functions outside of my tiny dark room laboratory; the beer also helped. Phil, thank you for allowing me to pursue tangential ideas which often lead nowhere. While a product may not exist, the knowledge acquired during iii the journey has become more valuable than success. I would also like to apologize for forcing you to spend ridiculous amounts of time correcting my spelilng and bad the grammer. To the professors with whom I had the pleasure of taking a class, both in the School of Earth and Space Exploration, as well as the School of Electrical, Computer, and Energy Engineering, thank you. I will never be able to adequately convey the joy I experience applying the knowledge shared in the classroom, to real-world problems. Special thanks to Danielle Bliss whose classes and advising dissipated my fear of complex mathematics. Thank you, Sam Gordon. The time spent devising weird ideas with you was pure joy. I am hopeful that in the near future we will finally figure out a way to talk to dolphins and translate dreams to video. Adrian Sinclair, thank you for mentoring me during my stay at ASU. Matthew Underhill, our morning coffee time will be forever cherished. If I had just half of your work ethic, I would have graduated in half the time. Jonathan Hoh, Cassandra Whitton, Edward Buie II, Marko best-DM-ever Neric, Kevin Hubbard, Rick Sarmento and Emily Lunde, our Tuesday Vine outing help to break up the monotony and aided in preserving my sanity. Lastly, thank you Jordyn Fani for putting up with me for the last decade. Your love, support, and assurance helped me become a scientist and a well-rounded person. I would not have been able to do this without you. This work has been funded by the NSF (Grant No. 1616906). iv TABLE OF CONTENTS Page LIST OF TABLES . ix LIST OF FIGURES . x CHAPTER 1 INTRODUCTION . 1 1.1 Motivations . 1 1.2 Resolution, Coherence, and Interference . 4 1.2.1 Spatial Resolution . 5 1.2.2 Coherent and incoherent wavefront . 7 1.2.3 Interference of light: classical wave formulation. 9 1.3 Classical Intensity Interferometry . 11 1.4 Modern Amplitude Interferometry . 16 2 INTENSITY INTERFEROMETRY AND SINGLE PHOTON TIMING . 22 2.1 Intensity Interferometry with Photomultiplier Tubes. 22 2.2 Intensity Interferometry with Single Photon Time Tagging . 25 2.3 Choice of Detectors . 28 2.4 Higher Order Correlation and Closure Phase . 31 2.5 Scalability of the telescope array . 33 2.6 UV -plane Exploration Due to Earths Rotation. 34 3 PHOTON STATISTICS . 37 3.1 Quantum of photon bunching . 38 3.2 Photon Occupation Number and Coherence Time . 41 3.3 Signal to Noise Ratio of Coherent, and False Positive Photons . 42 3.4 Signal to noise from stellar magnitude . 45 3.4.1 From Bessel calibration . 45 v CHAPTER Page 3.4.2 Comparing and Converting ηocc to Bessell Calibration for SNR Calculation . 46 3.5 Signal to Noise Ratio in Higher Order Correlations . 50 4 FROM THE STARS TO DATA . 52 4.1 Viable Targets From the Kitt Peak Observatory. 53 4.2 UV -plane Coverage from Kitt Peak . 57 4.3 Time bin shift . 63 4.4 Synchronization of the Decoupled Telescopes. 64 4.5 Optimal Telescope Size and the Affects of Seeing . 66 4.6 Telescope to SPAD coupling . 67 4.7 Readout Electronics. 69 5 READOUT ELECTRONICS AND DATA ANALYSIS . 73 5.1 Background . 73 5.2 Hardware . 74 5.2.1 ROACH2 FPGA Board . 74 5.2.2 LVTTL to LVDS Z-Dok Board . 75 5.3 Firmware . 77 5.3.1 Input filter . 77 5.3.2 Delay line . 79 5.3.3 Bubble filter . 81 5.3.4 Encoder . 81 5.3.5 Ping Pong Buffer . 83 5.4 Software . 84 5.5 Post-processing . 85 vi CHAPTER Page 6 LABORATORY TESTS . 88 6.1 Timing Precision . 88 6.2 Optical Setup . 90 6.3 RF Pickup and Modulation . 93 6.4 Hammond Box and RF Shielding . 96 6.5 Additional Peaks and Troughs . 98 7 IMAGE INVERSION SINGLE PHOTON INTERFEROMETRY FOR EXOPLANET CHARACTERIZATION . 100 7.1 General Overview . 100 7.2 Background . 102 7.2.1 Current Imaging Techniques . 103 7.2.2 Sub-Rayleigh resolution . 104 7.3 Semiclassical Explanation . 106 7.3.1 Theoretical limit and total cancellation of the host star light 106 7.3.2 Coherence time and single photon detection . 108 7.3.3 Associated Errors .
Details
-
File Typepdf
-
Upload Time-
-
Content LanguagesEnglish
-
Upload UserAnonymous/Not logged-in
-
File Pages168 Page
-
File Size-