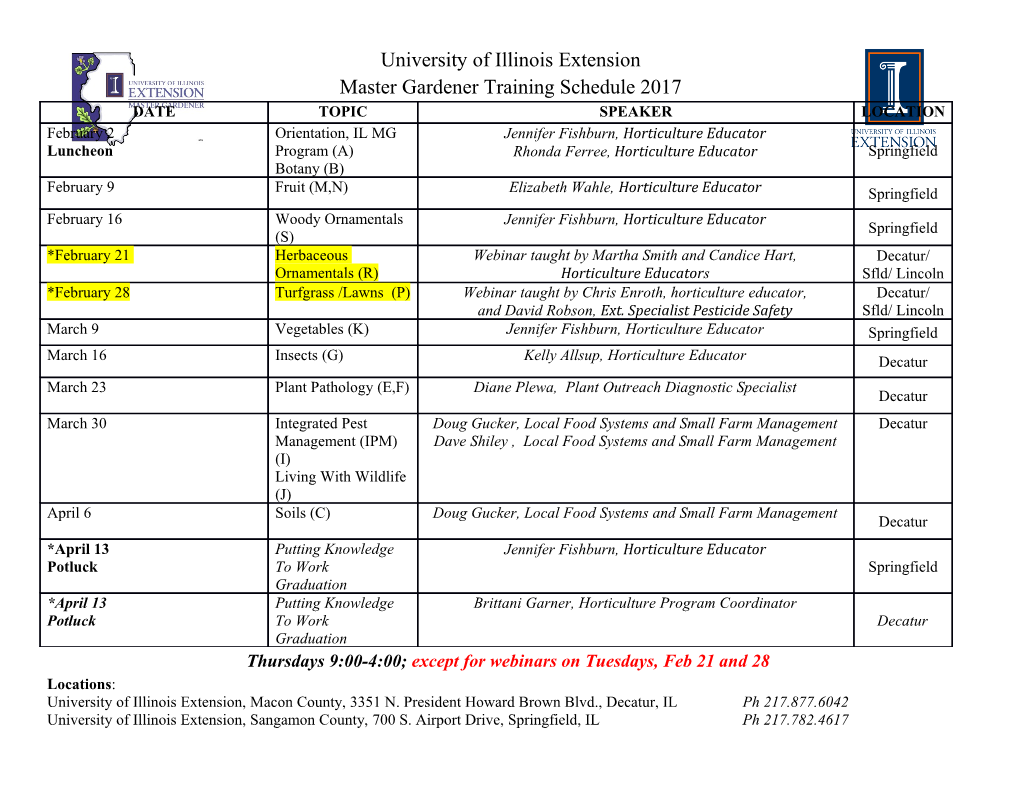
On Generalized Integrals and Ramanujan-Jacobi Special Functions N.D.Bagis Department of Informatics, Aristotele University Thessaloniki, Greece [email protected] Keywords: Elliptic Functions; Ramanujan; Special Functions; Continued Fractions; Generalization; Evaluations Abstract In this article we consider new generalized functions for evaluating integrals and roots of functions. The construction of these general- ized functions is based on Rogers-Ramanujan continued fraction, the Ramanujan-Dedekind eta, the elliptic singular modulus and other similar functions. We also provide modular equations of these new generalized functions and remark some interesting properties. 1 Introduction Let ∞ η(τ)= eπiτ/12 (1 e2πinτ ) (1) − n=1 Y denotes the Dedekind eta function which is defined in the upper half complex plane. It not defined for real τ. Let for q < 1 the Ramanujan eta function be | | ∞ arXiv:1309.7247v3 [math.GM] 15 Nov 2015 f( q)= (1 qn). (2) − − n=1 Y The following evaluation holds (see [12]): 1/3 1/2 1/24 1/12 1/3 1/2 f( q)=2 π− q− k k∗ K(k) (3) − 2 where k = kr is the elliptic singular modulus, k∗ = √1 k and K(x) is the complete elliptic integral of the first kind. − The Rogers-Ramanujan continued fraction is (see [8],[9],[14]): q1/5 q1 q2 q3 R(q) := ... (4) 1+ 1+ 1+ 1+ 1 which have first derivative (see [5]): 1 5/6 4 6 5 5 R′(q)=5− q− f( q) R(q) R(q) 11 R(q) (5) − − − − and also we can write p dR(q) 1 1/3 2/3 6 5 5 =5− 2 (kk∗)− R(q) R(q) 11 R(q) . (6) dk · − − − p Ramanujan have proven that 6 5 5 f( q) R(q)− 11 R(q) = − (7) − − qf( q5)6 − Also holds the following interesting identity 2 2 dk 2k(k∗) K(k) = − (8) dq qπ2 which is a result of Ramanujan (see [7] and [8]) for the first derivative of k = kr π√r with respect to q = e− , r> 0. 2 Propositions Definition 1. For any smooth function G, we define mG(A) to be such that + ∞ 4 πt A = π η (it/2) G R e− dt (9) Z√mG(A) Theorem 1. If y G(t) 5 dt = A (10) 6 5 5 0 t√t 11 t Z − − − then π√mG(A) y = R e− (11) Proof. From [5] it is known that if a,b (0, 1) then ∈ a R(a) 4 5/6 G(x) f( q) q− G(R(q))dq =5 dx. (12) 6 5 5 b − R(b) x√x 11 x Z Z − − − which is equivalent to write πa 1 a1 R(e− ) tπ 4 tπ/6 πt G(x) π f e− e− G R e− dt =5 dx. πb 6 5 5 − − 1 x√x 11 x Zb1 ZR(e− ) − − − 2 Setting b1 =+ , a1 = mG(A) and then using Definition 1 and the Dedekind eta expansion (1)∞ we get the result. p Also differentiating (8) one can get ( 1) 4 dmG− (r) πη (i√r/2) G(R(q)) π√r = , q = e− , r> 0 (13) dr − 2√r and if 2√r φ(r) := 4 (14) − i√r πη 2 G (R (q)) then Proposition 1. If A> 0, then d m (A)= φ (m (A)) (15) dA G G π√mG Derivating (10) with respect to qmG = e− we get 5G(R(q )) dR(q ) dA 1 mG mG = 6 5 5 dqmG R(qmG ) R(qmG )− 11 R(qmG ) dqmG dkmG − − dkmG p or the equivalent, using (8),(5) and (3): Theorem 2. dA G (y(A)) √3 = 2 2/3 (16) dkmG kmG km∗ G Also with integration of (16) we have A 3 1 1 7 2 1 3 2kmG 2F1 , ; ; kmG = dt (17) · 6 3 6 π√mG(t) 0 G R e− p Z Hence Definition 2. We define the function h as h(x) dt h(x) dt x = = (18) π√mG(t) G (y(t)) Z0 G R e− Z0 3 Theorem 3. Set mG(A)= r, then 1 1 7 A = h 3 3 2k F , ; ; k2 , (19) r · 2 1 6 3 6 r p or beter ( 1) 3 1 1 7 2 m − (r)= h 3 2k F , ; ; k . (20) G r · 2 1 6 3 6 r p From relation (10) differentiating we have G(y(A))y (A) 5 ′ =1 y(A) 6 y(A) 5 11 y(A)5 − − − hence p y(A) dt A dt 5 = = bmG (21) 6 5 5 0 t√t 11 t 0 G(y(t)) Z − − − Z where 1 1 7 b =3 3 2k F , ; ; k2 . (22) r r · 2 1 6 3 6 r p Also if m(x) is the function defined as (see [13]) + ∞ π η(it/2)4dt = r (23) Z√m(r) then ( 1) br− = m(r) (24) Hence π√mG y(A)= R e− = F1 (bmG ) (25) where we have define F1 by F1(x) dt x =5 . (26) 6 5 5 0 t√t 11 t Z − − − Theorem 4. A G(t)dt A dt 5 = h 5 (27) 6 5 5 6 5 5 0 t√t− 11 t 0 t√t− 11 t ! Z − − Z − − Proof. From relations (21) and (19) and (10) we have y(A) dt y(A) G(t)dt h 5 = h(bmG )= A =5 (28) 6 5 5 6 5 5 0 t√t− 11 t ! 0 t√t− 11 t Z − − Z − − 4 which by inversion of y(A) we get the desired result. Also from relation (20) is ( 1) mG− (A)= h(bA). (29) Hence knowing the function h we know almost everything. For this, for a given function P (x) we can set 1 6 5 5 G(x)=5− xP ′(x) x 11 x (30) − − − then p ( 1) π√A mG− (A)= P R e− = h(bA) (31) ( 1) Hence inverting bA we get the function h. Note also that P (A)= y − (A) and mG(A)= vi(y(A)) (32) π√x where vi(x) is the inverse function of R(e− ). Remarks. ( 1) 1) Note by definition that when we know G the mG− (A) is a closed form for- mula and mG(A) is not (it needs inversion). ( 1) 2) Continuing from relation (30) and y(x)= P − (x) we have 1 ( 1) ( 1) 6 ( 1) 5 ( 1) 5 G(y(x))=5− P − (x)P ′ P − (x) P (x) − 11 P (x) − − − − q From equation (28) we get also Corollary 1. y(A) ( 1) dt h − (A)=5 (33) 6 5 5 0 t√t 11 t Z − − − Corollary 2. 1 6 5 5 y′(A)=5− h′ (A)y(A) y(A) 11 y(A) (34) i − − − with p ( 1) h (A)= h − (A)= b = (b m ) (A) (35) i mG(A) ◦ G and also dy(A) 2/3 1 1/3 − 6 5 5 =5− 2 kmG km∗ G y(A) y(A)− 11 y(A) (36) dkmG · − − p Using the above one can show with differentiation the following 5 Theorem 5. y(A) ∞ 4 dt hi(A)= π η (it/2) dt = b G =5 (37) m (A) 6 5 5 √mG(A) 0 t√t− 11 t Z Z − − Also another interesting theorem arises from the definition of h(x). By setting ( 1) 1 − h (t) := (t), (38) 1 h (x) i′ then Theorem 6. Gi(x) dt x h (t) 5 = 1′ dt. (39) 6 5 5 0 t√t 11 t c t Z − − − Z Proof. From relation (18) we have 1 G (y(t)) = hi′ (t) hence inverting yi (Gi(t)) = h1(t). Taking the derivatives in both parts we get G (t) h (t) 5 i′ = 1′ . G (t) 6 G (t) 5 11 G (t)5 t i i − − − i Lastly integrating the abovep relation in both parts we get the result. Equation (39) can also written in the next form using (26) x h (t) G (x)= F 1′ dt (40) i 1 t Zc Suppose the n-th order modular equation of y(A) is ( 1) P (A)= y n y − (A) . (41) n · Then we can find Pn(x) by solving Pn(A) G(t) A G(t) dt = n dt (42) 6 5 5 6 5 5 0 t√t 11 t 0 t√t 11 t Z − − − Z − − − 6 with respect to Pn(A). Setting Qn(A) to be 2 ( 1) Q (A) := m n m − (A) (43) n G · G the n-nth degree modular equation of mG(A) and using (15) we have A ( 1) dt m − (A)= (44) G φ(t) Z0 and Qn(A) dt A dt = n . φ(t) φ(t) Z0 Z0 Also ( 1) Qn− (A)= Q1/n (A) and Qnm(A) dt Qm(A) dt A dt = n = nm φ(t) φ(t) φ(t) Z0 Z0 Z0 and Qn(Qm(A)) dt Qm(A) dt A dt = n = nm φ(t) φ(t) φ(t) Z0 Z0 Z0 Hence Qn(Qm(A)) dt Qnm(A) dt = φ(t) φ(t) Z0 Z0 and consequently Qn (Qm (A)) = Qm (Qn (A)) = Qnm(A), (45) a1 a2 as Q1(A)= A and if n = p1 p2 ...ps Q (x) = (Q ... Q ) (Q ... Q ) ... (Q ... Q ) (46) n p1 ◦ ◦ p1 ◦ p2 ◦ ◦ p2 ◦ ◦ ps ◦ ◦ ps respectively iterated a1,a2,...,as times. Hence ( 1) ( 1) ( 1) π m − Qn mG mG(A) y m − m − Q m m (A) = R e− G ◦ ◦ ◦ = G ◦ G ◦ n ◦ G ◦ G p πn√mG(A) = R e− =Ωn (y(A)) But ( 1) ( 1) ( 1) 2 m − m − Q m m (A)= m − n m (A) = Q∗ (A) (47) G ◦ G ◦ n ◦ G ◦ G G · G n By this we lead to the following 7 Theorem 7.
Details
-
File Typepdf
-
Upload Time-
-
Content LanguagesEnglish
-
Upload UserAnonymous/Not logged-in
-
File Pages32 Page
-
File Size-