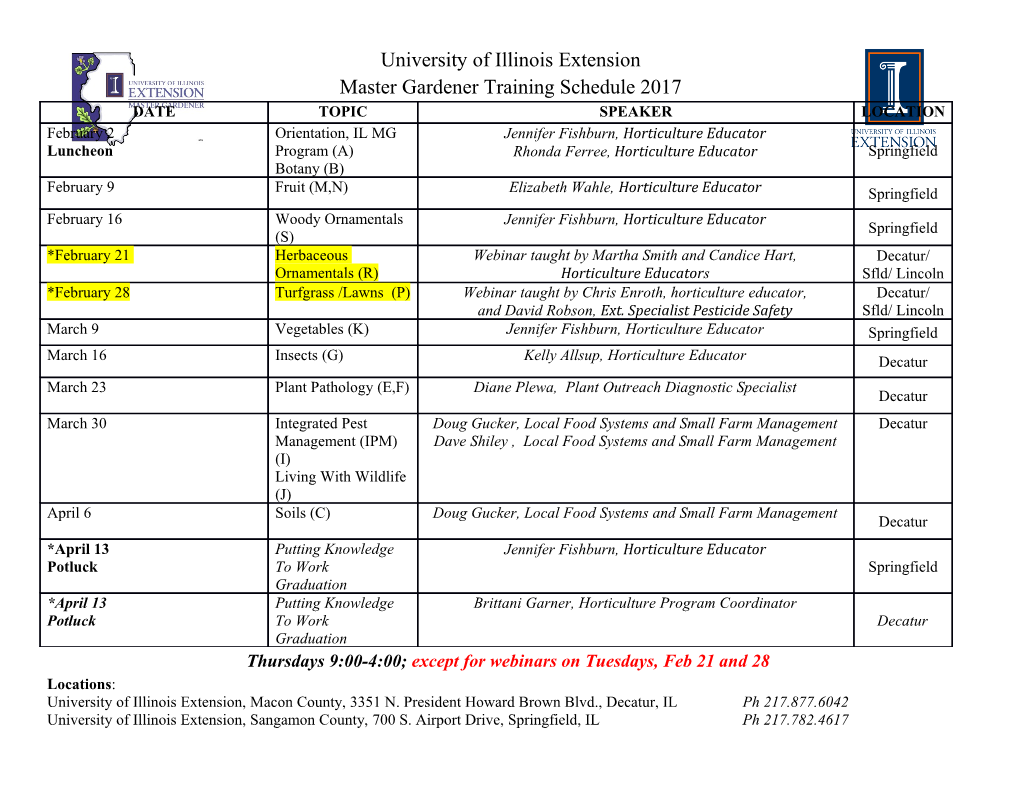
CMOS Signal Synthesizers for Emerging RF-to-Optical Applications Jahnavi Sharma Submitted in partial fulfillment of the requirements for the degree of Doctor of Philosophy in the Graduate School of Arts and Sciences COLUMBIA UNIVERSITY 2018 c 2017 Jahnavi Sharma All Rights Reserved ABSTRACT CMOS Signal Synthesizers for Emerging RF-to-Optical Applications Jahnavi Sharma The need for clean and powerful signal generation is ubiquitous, with applications spanning the spectrum from RF to mm-Wave, to into and beyond the terahertz-gap. RF applications including mobile telephony and microprocessors have effectively harnessed mixed-signal integration in CMOS to realize robust on-chip signal sources calibrated against adverse ambient conditions. Combined with low cost and high yield, the CMOS component of hand-held devices costs a few cents per part per million parts. This low cost, and integrated digital processing, make CMOS an attractive op- tion for applications like high-resolution imaging and ranging, and the emerging 5-G communication space. RADAR techniques when expanded to optical frequencies can enable micrometers of reso- lution for 3D imaging. These applications, however, impose upto 100x more exacting specifications on power and spectral purity at much higher frequencies than conventional RF synthesizers. This generation of applications will present unconventional challenges for transistor technologies - whether it is to squeeze performance in the conventionally used spectrum, already wrung dry, or signal generation and system design in the relatively emptier mm-Wave to sub-mmWave spectrum, much of the latter falling in the \Terahertz Gap". Indeed, transistor scaling and innovative device physics leading to new transistor topologies have yielded higher cut-off frequencies in CMOS, though still lagging well behind SiGe and III-V semiconductors. To avoid multimodule solutions with functionality partitioned across different technologies, CMOS must be pushed out of its comfort zone, and technology scaling has to have accompanying breakthroughs in design approaches not only at the system but also at the block level. In this thesis, while not targeting a specific application, we seek to formulate the obstacles in synthesizing high frequency, high power and low noise signals in CMOS and construct a coherent design methodology to address them. Based on this, three novel prototypes to overcome the limiting factors in each case are presented. The first half of this thesis deals with high frequency signal synthesis and power generation in CMOS. Outside the range of frequencies where the transistor has gain, frequency generation necessitates harmonic extraction either as harmonic oscillators or as frequency multipliers. We augment the traditional maximum oscillation frequency metric (fmax), which only accounts for transistor losses, with passive component loss to derive an effective fmax metric. We then present a methodology for building oscillators at this fmax, the Maximum Gain Ring Oscillator. Next, we explore generating large signals beyond fmax through harmonic extraction in multipliers. Applying concepts of waveform shaping, we demonstrate a Power Mixer that engineers transistor nonlinearity by manipulating the amplitudes and relative phase shifts of different device nodes to maximize performance at a specific harmonic beyond device cut-off. The second half proposes a new architecture for an ultra-low noise phase-locked loop (PLL), the Reference-Sampling PLL. In conventional PLLs, a noisy buffer converts the slow, low-noise sine-wave reference signal to a jittery square-wave clock against which the phase of a noisy voltage- controlled oscillator (VCO) is corrected. We eliminate this reference buffer, and measure phase error by sampling the reference sine-wave with the 50x faster VCO waveform already available on chip, and selecting the relevant sample with voltage proportional to phase error. By avoiding the N-squared multiplication of the high-power reference buffer noise, and directly using voltage- mode phase error to control the VCO, we eliminate several noisy components in the controlling loop for ultra-low integrated jitter for a given power consumption. Further, isolation of the VCO tank from any varying load, unlike other contemporary divider-less PLL architectures, results in an architecture with record performance in the low-noise and low-spur space. We conclude with work that brings together concepts developed for clean, high-power signal generation towards a hybrid CMOS-Optical approach to Frequency-Modulated Continuous-Wave (FMCW) Light-Detection-And-Ranging (LIDAR). Cost-effective tunable lasers are temperature- sensitive and have nonlinear tuning profiles, rendering precise frequency modulations or 'chirps' untenable. Locking them to an electronic reference through an electro-optic PLL, and electronically calibrating the control signal for nonlinearity and ambient sensitivity, can make such chirps possible. Approaches that build on the body of advances in electrical PLLs to control the performance, and ease the specification on the design of optical systems are proposed. Eventually, we seek to leverage the twin advantages of silicon-intensive integration and low-cost high-yield towards developing a single-chip solution that uses on-chip signal processing and phased arrays to generate precise and robust chirps for an electronically-steerable fine LIDAR beam. Table of Contents List of Figures v List of Tables xv Acknowledgement xvi Dedication xvii 1 Introduction 1 2 THz Frequency Synthesis: Maximum Gain Ring Oscillator 9 2.1 Technologies for high frequency signal generation . .9 2.2 45nm SOI CMOS Technology Characterization . 12 2.2.1 Active Devices . 12 2.2.2 Transmission Line . 15 2.2.3 Capacitor (VNCAP) . 16 2.3 Maximum Gain Ring Oscillator Topology . 17 2.3.1 Accounting for Passive Element Loss . 21 2.3.2 Determining the Matching Network . 24 2.3.3 Circuit-Level Implementation . 26 2.4 Harmonic Power Extraction and Spurious Mode Suppression . 29 2.4.1 Extracting the Kth harmonic . 29 2.4.2 Increasing the Output Power . 31 2.4.3 Maximizing the harmonic output power . 33 i 2.4.4 Suppression of spurious modes . 33 2.5 Oscillator Measurement . 36 2.6 Conclusion . 39 3 THz Power Generation: Frequency Multipliers 42 3.1 Scaling Trends in CMOS Multipliers . 42 3.2 A 134 GHz Doubler in 130 nm CMOS . 47 3.3 Conclusion . 49 4 THz Power Generation:Power Mixers 51 4.1 Concept of Nonlinearity Engineering in beyond-fmax Power Mixers.......... 53 4.1.1 Waveform shaping and output harmonic current . 54 4.1.2 Harmonic output power . 58 4.1.3 Input power requirement . 59 4.1.4 Effect of non-ideal input and output terminations . 61 4.2 180 − 200 GHz 130 nm CMOS Power Mixer Implementation . 64 4.2.1 130 nm CMOS 180 − 200 GHz Power Mixer . 65 4.2.2 130 nm CMOS 120 GHz Frequency Doubler . 67 4.2.3 130 nm CMOS Fundamental-frequency V-band PAs . 67 4.2.4 60 GHz Reflection-Type Phase Shifter (RTPS) . 69 4.2.5 60 GHz Variable Gain Amplifier . 75 4.2.6 60 GHz Marchand Balun . 77 4.3 Measurement . 79 4.3.1 60 GHz RTPS and VGA Breakout . 79 4.3.2 120 GHz Frequency Doubler Breakout . 81 4.3.3 180 − 200 GHz Power Mixer . 83 4.4 Conclusion . 87 5 Low Noise and Low Spur RF PLL: Reference-Sampling PLL 91 5.1 Review . 91 5.1.1 Conventional Type-II Second Order PLLs . 92 ii 5.1.2 Sub-sampling PLLs . 94 5.1.3 Injection-Locked Clock Multipliers (ILCM) . 101 5.2 New Sampled RF-PLL approach: Reference-Sampling Phase Locked Loop (RSPLL) 105 5.2.1 Motivation: Low noise and Low Spur . 105 5.2.2 Sampled Phase detector (PD) . 107 5.2.3 Sample Edge Selection Circuit (SESCi) . 112 5.2.4 Frequency Tracking Loop . 114 5.2.5 Proposed PLL Architecture . 114 5.2.6 Noise and Power Analysis . 116 5.2.7 Effect of nonidealities . 125 5.3 Proposed Loop Implementation . 130 5.3.1 Loop parameter selection . 130 5.3.2 Switch size . 132 5.3.3 SESCi . 132 5.3.4 Reference Buffer . 133 5.3.5 LC-VCO Implementation . 136 5.3.6 VCO Buffer . 137 5.3.7 Frequency Tracking Loop . 137 5.3.8 Output Test Buffer . 140 5.3.9 Ground isolation and ESD protection . 140 5.4 Simulated performance and Measurement . 141 5.4.1 Phase noise simulation . 141 5.4.2 Measured Performances: VCO . 145 5.4.3 Measured Performances: RSPLL . 145 5.5 Comparison . 148 5.6 Future work: Loop Bandwidth Modification . 149 6 Wide Bandwidth Electro-optic PLLs for FMCW LIDAR 152 6.1 Theory of FMCW detection . 153 6.2 Photo-electric interface . 157 6.3 EO-PLL Basics . 158 iii 6.4 Proposed EO-PLL for FMCW LIDAR . 162 6.4.1 Motivation: Reduction in MZI delay and implementation area . 162 6.4.2 Loop architecture . 164 6.4.3 Implementation . 164 6.4.4 Acquisition of mixer-based phase detector . 170 6.4.5 Measured Perfomance . 170 6.5 Future work . 171 6.5.1 Mixer-based EO-PLL . 171 6.5.2 Conventional EO-PLL around a Laser Phase Shifter . 174 7 Conclusion 178 I Bibliography 181 Bibliography 182 II Appendices 197 A Effect of Oscillator Non-ideality in OFDM 198 A.1 Inter-carrier interference . 198 A.2 Phase Noise . 200 B Race Conditions in Multi-loop PLLs 203 iv List of Figures 2 1.1 Phase noise and FoM = fc = (L P ) for state-of-the-art CMOS PLLs PN ∆fc ∆fc DC;mW for different carrier frequencies fc at different offsets ∆fc. Maximum phase noise limits for m-QAM at the OFDM-based standard's carrier spacing is shown for PLLs with loop bandwidth of 100 kHz. 3GPP to WiGig impose phase noise requirements at increasing carrier spacing ∆fc. Requirements for evolving OFDM-based standards are met by CMOS for at least 64 QAM, but with poorer FOMPN ...........2 1.2 Output power and efficiency roll-off in CMOS with increasing frequency.
Details
-
File Typepdf
-
Upload Time-
-
Content LanguagesEnglish
-
Upload UserAnonymous/Not logged-in
-
File Pages227 Page
-
File Size-