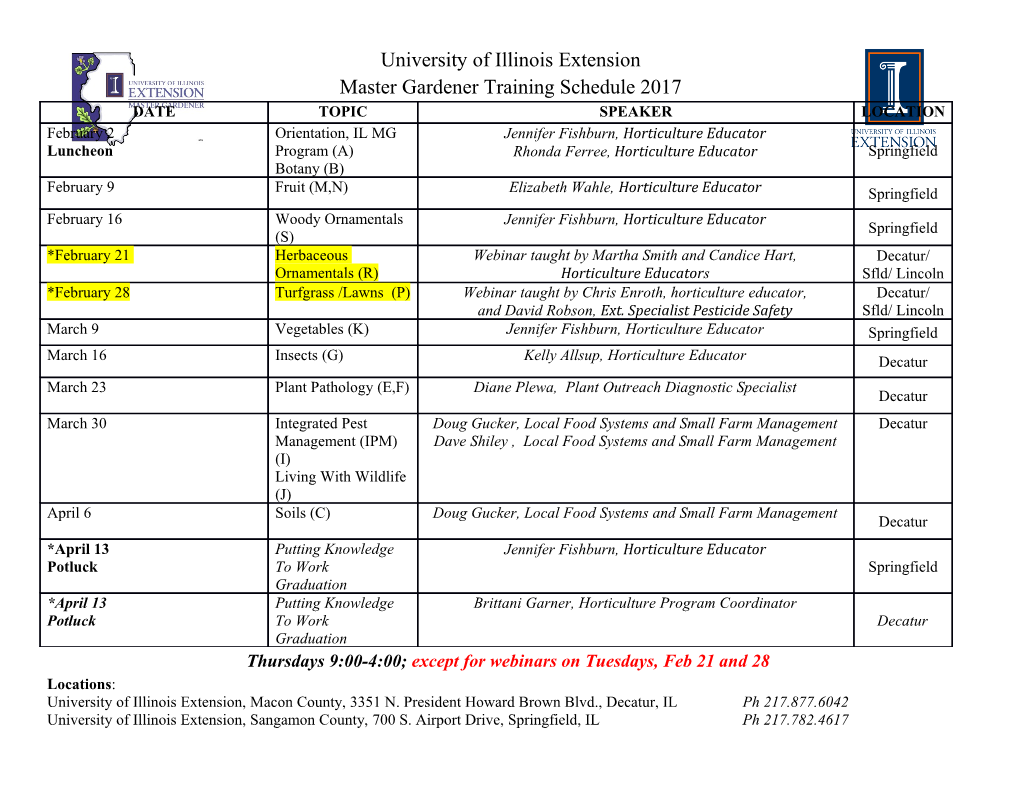
biomolecules Review TGF-β Signaling Kalliopi Tzavlaki and Aristidis Moustakas * Department of Medical Biochemistry and Microbiology, Science for Life Laboratory, Uppsala University, Box 582, SE-751 23 Uppsala, Sweden; [email protected] * Correspondence: [email protected]; Tel.: +46-18-4714732; Fax: +46-18-4714441 Received: 18 February 2020; Accepted: 20 March 2020; Published: 23 March 2020 Abstract: Transforming growth factor-β (TGF-β) represents an evolutionarily conserved family of secreted polypeptide factors that regulate many aspects of physiological embryogenesis and adult tissue homeostasis. The TGF-β family members are also involved in pathophysiological mechanisms that underlie many diseases. Although the family comprises many factors, which exhibit cell type-specific and developmental stage-dependent biological actions, they all signal via conserved signaling pathways. The signaling mechanisms of the TGF-β family are controlled at the extracellular level, where ligand secretion, deposition to the extracellular matrix and activation prior to signaling play important roles. At the plasma membrane level, TGF-βs associate with receptor kinases that mediate phosphorylation-dependent signaling to downstream mediators, mainly the SMAD proteins, and mediate oligomerization-dependent signaling to ubiquitin ligases and intracellular protein kinases. The interplay between SMADs and other signaling proteins mediate regulatory signals that control expression of target genes, RNA processing at multiple levels, mRNA translation and nuclear or cytoplasmic protein regulation. This article emphasizes signaling mechanisms and the importance of biochemical control in executing biological functions by the prototype member of the family, TGF-β. Keywords: extracellular matrix; phosphorylation; receptor serine/threonine kinase; signal transduction; SMAD; transcription; transforming growth factor-β; ubiquitylation 1. Introduction Biological signals regulate every aspect of physiological development of multicellular organisms and are important for the communication and coordination of cellular, tissue, and organ functions throughout life [1]. Among the large number of signaling molecules, the transforming growth factor β (TGF-β) family is highly conserved in the animal kingdom, and is thought to have appeared from the early days of multicellular (metazoan) evolution [2–6]. Across many species, the TGF-βs mediate a diverse range of embryonic and adult signaling functions that provide tissue-specific control of differentiation, proliferation, and cell-specific or tissue-specific motility [7–10]. The human TGF-β family includes thirty-three genes that encode for homodimeric or heterodimeric secreted cytokines [10,11]. These proteins are synthesized in a precursor form that is cleaved during processing through the secretory pathway and generate mature dimeric ligands most often held together via a single disulfide bond [11,12]. The family members have received a variety of names based on the history of their molecular identification, and include the activins, the bone morphogenetic proteins (BMPs), the growth differentiation factors (GDFs), the müllerian inhibiting substance (MIS), the nodal and the TGF-βs. Due to space limitation, this article will focus on the three TGF-βs (TGF-β1, -β2, -β3), which are collectively referred as TGF-β, and with occasional references to other family members. Like in every other signaling network, regulation at multiple levels is of paramount importance so that the pathways operate physiologically and perform their normal function [1]. Genetic mutations Biomolecules 2020, 10, 487; doi:10.3390/biom10030487 www.mdpi.com/journal/biomolecules Biomolecules 2020, 10, 487 2 of 38 can occur in several of the central molecular mediators of TGF-β signaling [8,9]. In addition, and more frequently, dedicated regulators of TGF-β family pathways malfunction, either due to their misexpression or due to genetic mutation, leading to weak or more often enhanced signaling input by the TGF-β signaling engine [8–10]. Such perturbations associate with the onset or alternatively with late stages of different diseases that include fibrotic disorders, chronic inflammatory conditions, and cancer [8,9]. In this article, the discussion will not focus on disease and only occasionally, examples from the pathophysiology of various diseases may be used, to illustrate control of signal transduction. 2. TGF-β Synthesis, Extracellular Deposition, and Activation The elucidation of crystal structures for the various TGF-β family members, coupled to detailed biochemical and biophysical studies have shed light to the importance of TGF-β ligand processing, extracellular deposition and the mechanisms of their activation for presentation to signaling receptors [13,14]. We will now review this well-researched topic of TGF-β biogenesis and activation. Similar to all other secreted proteins, TGF-β is synthesized by ribosomes attached to the rough endoplasmic reticulum of most cells, where removal of the short N-terminal signal peptide allows protein folding, glycosylation, and processing in subsequent biosynthetic steps during transport from the endoplasmic reticulum to the Golgi apparatus (Figure1)[ 12,14]. TGF-β protein folding is intimately connected to the formation of intermolecular disulfide bonds, two in the N-terminal region that will later become the long prodomain and one in the C-terminal region that will later be the short mature ligand, resulting in obligatory dimerization of the unprocessed ligand (Figure1)[ 12]. Glycosylation of the N-terminal part of the polypeptide is known to confirm latency, i.e., inactivity of the newly synthesized TGF-β [15], leading to the concept that functional activation is required at a later stage. Dimerization via disulfide linkage is followed by proteolytic cleavage of the polypeptides by furin family proteases, resulting in the formation of an N-terminal long dimeric and disulfide-linked propeptide, also known as latency-associated peptide (LAP), and a C-terminal short dimeric disulfide-linked polypeptide, also known as mature TGF-β (Figure1)[ 10,12]. The two parts of TGF-β, generated after proteolytic cleavage, the LAP and the mature TGF-β, remain associated with each other and form the latent form of the ligand, often referred as large latent complex (LLC, Figure1), whereby latency means lack of direct biological activity in the absence of further processing. Structural analysis of the latent form of TGF-β has elucidated the detailed molecular mechanism by which LAP directly covers the critical amino acids of the C-terminal dimer that are later used for interaction with the signaling receptors, and thus confers inactivation of the C-terminal dimer, when assembled as a latent complex [13]. It is of interest to consider the fact that during animal evolution, this principle of “masking” the mature dimeric ligand by the N-terminal part of the pro-peptide (LAP), in other members of the TGF-β family, has diverged so that the inhibitory polypeptides are actually expressed by a completely distinct gene. A good example of this difference is the inhibitory protein noggin that binds and inactivates a group of the BMPs [13]. Concomitant to the processing of the TGF-β polypeptide, crosslinking of the N-terminal LAP to other secreted proteins takes place (Figure1). Two major families of proteins directly crosslink to the latent form of TGF-β in a cell type-specific or biological context-dependent manner; these are the latent TGF-β binding proteins (LTBPs) and the leucine rich repeat containing (LRRC) 32/33 proteins [14,16,17]. The LTBPs (such as LTBP1 and LTBP3 which interact with all three LAP-TGF-β1, -2, -3; LTBP4, which interacts with LAP-TGF-β1; LTBP2 which interacts with pro-myostatin/pro-GDF-8) are extracellular proteins, and upon secretion, mediate deposition of latent TGF-βs to the extracellular matrix (ECM) [14]. Via their ability to crosslink with additional proteins of the ECM, e.g., fibrillins and fibronectins, LTBPs provide the scaffolding units that tether latent TGF-βs to the ECM (Figure1)[14]. The second group of latent TGF-β crosslinkers, the LRRC32/33 proteins are transmembrane proteins. LRRC32, also known as glycoprotein A repetitions predominant (GARP), is primarily expressed in immune cells, such as regulatory T cells (Treg) and crosslinks to latent TGF-β [16]. Similarly, LRRC33, primarily expressed in the plasma membrane of immune modulators of the brain, the microglial cells, Biomolecules 2020, 10, 487 3 of 38 also tethers latentBiomolecules TGF- 2020β, 10[,17 x ]. Whether incorporated into the complex ECM environment3 of 38 or associated with the plasmaenvironment membrane or associated of cells with that the regulateplasma membrane immunity, of cells thethat disulfide-linkedregulate immunity, the latent disulfide- TGF-βs depend on mechanismslinked of activationlatent TGF-βs anddepend release on mechanisms of their matureof activation dimeric and release ligands, of their as mature discussed dimeric below. ligands, as discussed below. 3´ 3´ 3´ 3´ Ribosome SRP C C C C 5´ N 5´ N 5´ N 5´ N Translocon SRP receptor Signal peptidase ER lumen Cytoplasm Monomer Dimer LLC C LTBP ER lumen N Secretory vesicle Trans-Golgi lumen LLC LLC C N Furin LLC C N C LLC LTBP C N N Exocytosis Cytoplasm LLC C ECM N N LLC LLC LLC N C N N N C C N N C Fibrillin N N C C Fibronectin C Fibrillin C Fibronectin C Figure 1. BiosynthesisFigure 1. Biosynthesis and extracellular and extracellular deposition deposition of of transforming
Details
-
File Typepdf
-
Upload Time-
-
Content LanguagesEnglish
-
Upload UserAnonymous/Not logged-in
-
File Pages38 Page
-
File Size-