Microelectrode Implants for Spinal Cord Stimulation in Rats
Total Page:16
File Type:pdf, Size:1020Kb
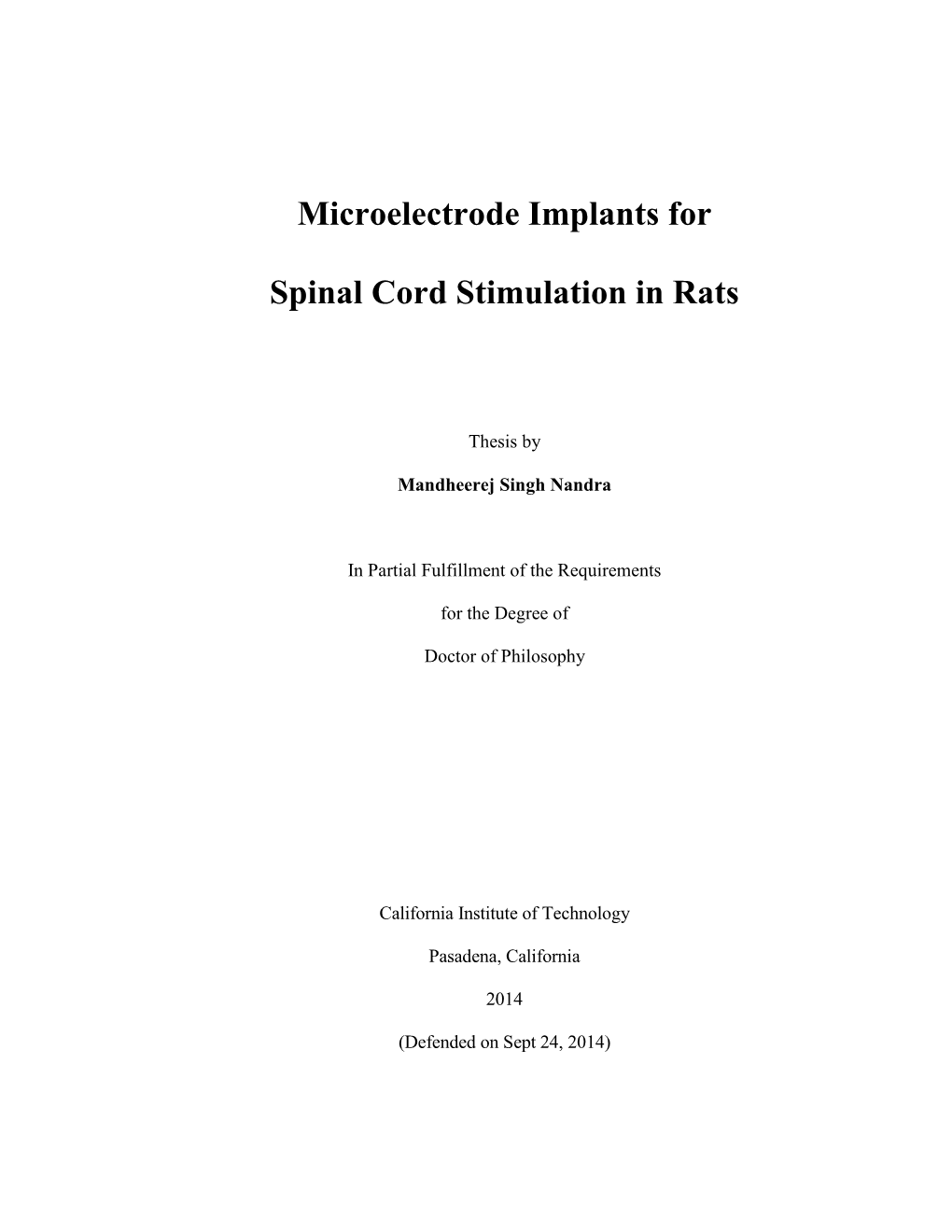
Load more
Recommended publications
-
Precision™ Spinal Cord Stimulator System Clinician Manual Directions for Use
Precision™ Spinal Cord Stimulator System Clinician Manual Directions for Use 91083273-04 CAUTION: Federal law restricts this device to sale, Content: 92162683 REV A distribution and use by or on the order of a physician. Precision™ Spinal Cord Stimulator System Clinician Manual Guarantees Boston Scientific Corporation reserves the right to modify, without prior notice, information relating to its products in order to improve their reliability or operating capacity. Drawings are for illustration purposes only. Trademarks All trademarks are the property of their respective holders. Clinician Manual 91083273-04 ii of iv Table of Contents Manual Overview ...........................................................................................................................1 Device and Product Description ..................................................................................................2 Implantable Pulse Generator ...........................................................................................................2 Leads ...............................................................................................................................................2 Lead Extension ................................................................................................................................2 Lead Splitter ......................................................................................................................................3 Indications for Use ........................................................................................................................4 -
Management of Chronic and Neuropathic Pain with 10 Khz Spinal Cord Stimulation Technology: Summary of Findings from Preclinical and Clinical Studies
biomedicines Review Management of Chronic and Neuropathic Pain with 10 kHz Spinal Cord Stimulation Technology: Summary of Findings from Preclinical and Clinical Studies Vinicius Tieppo Francio 1,* , Keith F. Polston 1 , Micheal T. Murphy 1 , Jonathan M. Hagedorn 2 and Dawood Sayed 3 1 Department of Rehabilitation Medicine, The University of Kansas Medical Center, Kansas City, KS 66160, USA; [email protected] (K.F.P.); [email protected] (M.T.M.) 2 Department of Anesthesiology and Perioperative Medicine, Division of Pain Medicine, Mayo Clinic, Rochester, MN 55905, USA; [email protected] 3 Department of Anesthesiology, The University of Kansas Medical Center, Kansas City, KS 66160, USA; [email protected] * Correspondence: [email protected] Abstract: Since the inception of spinal cord stimulation (SCS) in 1967, the technology has evolved dramatically with important advancements in waveforms and frequencies. One such advance- ment is Nevro’s Senza® SCS System for HF10, which received Food and Drug and Administration (FDA) approval in 2015. Low-frequency SCS works by activating large-diameter Aβ fibers in the lateral discriminatory pathway (pain location, intensity, quality) at the dorsal column (DC), creating paresthesia-based stimulation at lower-frequencies (30–120 Hz), high-amplitude (3.5–8.5 mA), and µ Citation: Tieppo Francio, V.; Polston, longer-duration/pulse-width (100–500 s). In contrast, high-frequency 10 kHz SCS works with a K.F.; Murphy, M.T.; Hagedorn, J.M.; proposed different mechanism of action that is paresthesia-free with programming at a frequency Sayed, D. Management of Chronic of 10,000 Hz, low amplitude (1–5 mA), and short-duration/pulse-width (30 µS). -
Neuropathic Pain Case
Author Information Full Names: Erica Patel, MD Kiran V. Patel, MD Presenting Symptom: Burning in right foot> Chronic low back pain Case Specific Diagnosis: Chronic low back pain and radicular pain Learning Objectives: 1. To identify the factors affecting failure of trials (<50% pain reduction in pain for trial period). 2. Demonstrate ways to improve the success of spinal cord stimulation (SCS) trial. 3. Discuss and review literature on SCS efficacy in treating various chronic pain syndromes. History: A 75 year old female retired librarian with a past medical history of DM, and history of L5-S1 laminotomy/microdiscectomy ten years ago who presents with chronic low back pain and right burning foot pain for the past year. In the past six months the pain has increased in intensity. She denies any recent falls or trauma. She denies any bladder or bowel incontinence, weight loss, fever, chills or weakness of her lower extremities. She is interested in minimally invasive interventions to treat her pain and wants to avoid surgery if possible. The pain occurs daily and begins in the middle of the low back and travels to the sole of the right foot associated with a burning sensation. This pain is affecting her quality of life. She is unable to walk for more than 15 minutes due to the worsening back and leg pain. Her sleep is fragmented secondary to the burning in the right foot. Aggravating factors include bending, walking, and sitting. Alleviating factors include a TENS unit and rest. She follows with her primary regularly and states her “diabetes -
Pain Management Centre Spinal Cord Stimulator Pathway
Pain Management Centre Spinal Cord Stimulator Pathway Patient Information Leaflet for: Neuromodulation Pathway Author/s: K Dyer, B Roughsedge, G Daniels Author/s titles: Clinical Nurse Manager, Clinical Psychologist, Specialist Physiotherapist Approved by: Patient Information Forum Date approved: 03/01/2019 Review date: 03/01/2022 Available via Trust Docs Version: 7 Trust Docs ID:10179 Pain Management Centre Spinal Cord Stimulator (SCS) Pathway Your Consultant has suggested that you might be suitable for a trial of spinal cord stimulation (SCS). National Institute for Health and Clinical Excellence (NICE) guidelines for SCS state that “spinal cord stimulation should be provided only after an assessment by a multidisciplinary team experienced in chronic pain assessment and management of people with spinal cord stimulation devices, including experience in the provision of ongoing monitoring and support of the person assessed.” (NICE 2008). The multi-disciplinary team comprises Consultants, Specialist Nurses, Clinical Psychologists, Occupational Therapist & Specialist Physiotherapists, all who have many years’ experience of managing chronic pain with spinal cord stimulation. We have developed a pathway to comply with this guidance, which will start when the consultant recommends you for assessment by the spinal cord stimulator multidisciplinary team. The Pathway - what happens next? The first appointment in the pathway is a Technical Session. This is a group appointment and you are welcome to bring a relative/ friend with you. During this session we will: • Explain what spinal cord stimulation is • Demonstrate the types of equipment used • Discuss the risks associated with the device • Discuss the potential benefit you may receive from SCS • Discuss the trial and post op instructions if you proceed You will also be given an appointment with two members of the multi-disciplinary team. -
Spinal Cord Stimulation: a Nonopioid Alternative for Chronic Pain Management
PRACTICE | INNOVATIONS CPD Spinal cord stimulation: a nonopioid alternative for chronic pain management Aaron Hong MD MSc, Vishal Varshney MD, Gregory M.T. Hare MD PhD, C. David Mazer MD n Cite as: CMAJ 2020 October 19;192:E1264-7. doi: 10.1503/cmaj.200229 hronic pain affects 1 in 5 Canadians and is associated 1 with considerable socioeconomic burden. Although opi- KEY POINTS oids have been the mainstay of treatment, they have lost Spinal cord stimulation masks pain signals through a Cfavourability owing to crises of addiction, abuse, tolerance and • transcutaneous implantable electric pulse generator. dependence.1,2 Consequently, alternatives — including cognitive • Spinal cord stimulation is safe, efficacious and cost-effective in behavioural therapy, physical rehabilitation, non-opiate pharma- chronic pain management of neuropathic pain conditions, 1,3 cology and integrative therapies — have been developed. including failed back surgery syndrome, chronic regional pain When conventional therapies produce unacceptable adverse syndrome and chronic peripheral neuropathies. effects or do not provide sufficient pain relief, spinal cord • Newer spinal cord stimulation technologies are expanding stimulation (neuromodulation) may offer a rescue option, either clinical indications such as visceral and ischemic pain, with alone or in conjunction with other modalities.3,4 potential for further improved efficacy. Neuromodulation, defined as the alteration of nerve activity • Increased awareness of and access to spinal cord through targeted stimulus delivery, was first introduced in stimulation therapy may allow more Canadians to benefit 2,3,5 from relief of intractable chronic pain and may reduce 1967. It is based on the principle of electrically stimulating the opioid consumption. -
1. Summary of Safety and Effectiveness Data
1. Summary Of Safety And Effectiveness Data 1.1 General Information 1.1.1 Device Generic Name Totally Implanted Spinal Cord Stimulator for Pain Relief 1.1.2 Device Trade Name Genesis Neurostimulation (IPG) System 1.1.3 Applicant’s Name and Address Advanced Neuromodulation Systems (ANS), Inc. 6501 Windcrest Drive, Suite 100 Plano, Texas 75024 1.1.4 PMA Number P010032 1.1.5 Date of Notice of Approval to the Applicant November 21, 2001 1.2 Indications for Use ANS Genesis Neurostimulation (IPG) System is indicated as an aid in the management of chronic intractable pain of the trunk and/or limbs, including unilateral or bilateral pain associated with the following: failed back surgery syndrome, intractable low back and leg pain. 1.3 Device Description 1.3.1 Genesis Neurostimulation System The Genesis Neurostimulation (IPG) System consists of the following components: Model 3608 Implanted Pulse Generator (IPG), Model 3850 Patient Programmer, Model 1232 Programming Wand, and Model 1210 Patient Magnet. The Genesis Neurostimulation (IPG) System is intended to be used with the following ANS’ legally marketed components: · percutaneous lead models 3143, 3146, 3153, 3156, 3183 and 3186 · surgical lead models 3222, 3240, 3244 and 3280 · extension models 3382, 3383, 3341, 3342 and 3343 · ANS TS8 test stimulation system. The IPG is connected to a lead with four or eight electrodes, either directly or with a lead extension. The electrodes contact the patient along the spinal cord. The IPG is implanted in a subcutaneous pocket, and receives radio frequency (RF) programming signals from an external Patient 1 of 17 Programmer. -
Is Spinal Cord Stimulation an Effective Therapy to Treat Severe Lower
Philadelphia College of Osteopathic Medicine DigitalCommons@PCOM PCOM Physician Assistant Studies Student Student Dissertations, Theses and Papers Scholarship 2018 Is spinal cord stimulation an effective therapy to treat severe lower extremity painful diabetic peripheral neuropathy that has lasted over one year and has not responded to medical therapy? Eleni Brecht Philadelphia College of Osteopathic Medicine Follow this and additional works at: https://digitalcommons.pcom.edu/pa_systematic_reviews Part of the Medicine and Health Sciences Commons Recommended Citation Brecht, Eleni, "Is spinal cord stimulation an effective therapy to treat severe lower extremity painful diabetic peripheral neuropathy that has lasted over one year and has not responded to medical therapy?" (2018). PCOM Physician Assistant Studies Student Scholarship. 357. https://digitalcommons.pcom.edu/pa_systematic_reviews/357 This Selective Evidence-Based Medicine Review is brought to you for free and open access by the Student Dissertations, Theses and Papers at DigitalCommons@PCOM. It has been accepted for inclusion in PCOM Physician Assistant Studies Student Scholarship by an authorized administrator of DigitalCommons@PCOM. For more information, please contact [email protected]. Is spinal cord stimulation an effective therapy to treat severe lower extremity painful diabetic peripheral neuropathy that has lasted over one year and has not responded to medical therapy? Eleni Brecht, PA-S A SELECTIVE EVIDENCE BASED MEDICINE REVIEW In Partial Fulfillment of the Requirements -
Spinal Cord Stimulators (Dorsal Column Stimulators)
Spinal Cord Stimulators (Dorsal Column Stimulators) Date of Origin: 06/2006 Last Review Date: 05/27/2020 Effective Date: 06/01/2020 Dates Reviewed: 07/2007, 10/2007, 10/2008, 07/2010, 07/2011, 07/2012, 05/2013, 07/2014, 03/2016, 06/2017, 08/2018, 05/2019, 05/2020 Developed By: Medical Necessity Criteria Committee I. Description Spinal cord stimulators, also known as dorsal column stimulators, deliver low voltage electrical stimulation to the dorsal columns of the spinal cord in order to block pain sensations. These devices consist of a lead that delivers the electrical stimulation to the spinal cord, an extension wire that conducts the electrical stimulation from the power source to the lead, and a power source which generates the electrical stimulation. Totally implantable spinal cord stimulators are most commonly used; however, there are also spinal cord stimulators which rely on radio frequency and include a transmitter and an antenna which are carried outside the body and a receiver, which is implanted inside the body. Implantation of the spinal cord stimulator is generally a two- step process. This process includes a trial period of stimulation in which an electrode is temporarily implanted in the epidural space. Once treatment is deemed effective, through a significant reduction in pain, the spinal cord stimulator is permanently implanted. Successful spinal cord stimulation may require extensive programming of the neurostimulators to identify the optimal electrode combinations and stimulation channels. Spinal cord stimulator placement is a non-destructive, reversible procedure and thus is often an attractive alternative for patients who have failed other treatment and surgical options. -
Spinal Cord Stimulation (SCS)
Spinal Cord Stimulation (SCS) A spinal cord stimulator (SCS) is a device, which delivers low voltage electrical stimulation to the spinal cord. The electrical stimulation to the spinal cord is delivered through one or two wires, which are carefully placed in the epidural space. The epidural space is a space adjacent to the spinal cord. Frequently asked Questions: 1. How does Spinal Cord Stimulation work? The electrical signals sent by the spinal cord stimulator replace the sensation of pain with a tingling sensation. 2. How is the procedure performed? The spinal cord stimulator is implanted in two stages. The first stage is a trial and it is performed in the operating room with local anesthetic and intravenous sedation. In this stage, we place the wires through the skin, into the epidural space, which is adjacent to the spinal cord. Then the wires are connected to a stimulating generator. We leave the wires in place for 3 to 7 days. This is a test to determine if a permanent stimulating generator should be used. If the trial is successful, we place a permanent generator under the skin. The generator contains a battery. When the battery runs down it is replaced. Placement of the generator under the skin is done in the operating room and, after the procedure, the patient goes home. General anesthesia is not needed for the implantation of the spinal cord stimulator. 3. What are the benefits of SCS? The goal of the implantation is to reduce rather than eliminate pain. It might reduce the pain by 50 percent or more, it could increase the patient’s activity level and it might reduce the use of narcotic medications. -
Spinal Cord Stimulation for Practitioners; an Introduction
Spinal Cord Stimulation for Practitioners; An Introduction Steven Falowski, MD Introduction Spinal cord stimulation(SCS) is an adjustable, non-destructive, neuromodulatory procedure which delivers therapeutic doses of electrical current to the spinal cord for the management of neuropathic pain. The most common indications include post-laminectomy syndrome, complex regional pain syndrome (CRPS), ischemic limb pain, and angina. Scattered reports regarding the treatment of intractable pain due to other causes including visceral/abdominal pain, cervical neuritis pain, spinal cord injury pain, post-herpetic neuralgia, and neurogenic thoracic outlet syndrome have also appeared in the literature. The procedures are most commonly performed by neurosurgeons or anesthesiologists specializing in pain management but other specialties, such as rehabilitation medicine and orthopedic surgery, have also demonstrated interest in the procedure. Relevant Anatomy Understanding the somatotopy of the spinal cord is paramount to knowing the technical aspects of implantation. A basic tenet of SCS is to create an overlapping of paresthesia and pain region. In order to do this, correlation of the somatotopy and the level of the spinal cord is necessary. Barolat has published extensively on the mapping of the spinal structures in man. A database was created to suggest areas of sensory response to dorsal spinal cord stimulation. High cervical regions such as C2 can cover the posterior occipital region, and occasionally the lower jaw. C2-4 stimulation will provide coverage of the shoulder while stimulation in the lower cervical region such as C5-6 will provide for the entire hand. To cover the anterior chest wall or the axilla, an electrode towards C7 will be necessary. -
Cigna CMM-211 Spinal Cord Stimulators
Cigna Medical Coverage Policies – Musculoskeletal Spinal Cord and Dorsal Root Ganglion Stimulation Effective January 15, 2020 ___________________________________________________________________________________________ Instructions for use The following coverage policy applies to health benefit plans administered by Cigna. Coverage policies are intended to provide guidance in interpreting certain standard Cigna benefit plans and are used by medical directors and other health care professionals in making medical necessity and other coverage determinations. Please note the terms of a customer’s particular benefit plan document may differ significantly from the standard benefit plans upon which these coverage policies are based. For example, a customer’s benefit plan document may contain a specific exclusion related to a topic addressed in a coverage policy. In the event of a conflict, a customer’s benefit plan document always supersedes the information in the coverage policy. In the absence of federal or state coverage mandates, benefits are ultimately determined by the terms of the applicable benefit plan document. Coverage determinations in each specific instance require consideration of: 1. The terms of the applicable benefit plan document in effect on the date of service 2. Any applicable laws and regulations 3. Any relevant collateral source materials including coverage policies 4. The specific facts of the particular situation Coverage policies relate exclusively to the administration of health benefit plans. Coverage policies are not recommendations for treatment and should never be used as treatment guidelines. This evidence-based medical coverage policy has been developed by eviCore, Inc. Some information in this coverage policy may not apply to all benefit plans administered by Cigna. CPT® (Current Procedural Terminology) is a registered trademark of the American Medical Association (AMA). -
Outcomes and Predictors of Revisions of Spinal Cord Stimulators
NEUROSURGICAL FOCUS Neurosurg Focus 40 (5):E4, 2016 Neuromodulation in intractable pain management: outcomes and predictors of revisions of spinal cord stimulators Shyamal C. Bir, MD, PhD, Subhas Konar, MCh, Tanmoy Maiti, MD, MCh, Anil Nanda, MD, MPH, and Bharat Guthikonda, MD Department of Neurosurgery, Louisiana State University Health–Shreveport, Louisiana OBJECTIVE Spinal cord stimulators (SCSs) appear to be safe and efficacious for chronic intractable back pain. Al- though there are many reports on percutaneous SCSs, there are very few studies on outcomes of paddle lead SCSs. In addition, the predictors of requirement for SCS revision have not been well established. Here, the authors review the outcome of a case series and attempt to identify the predictors of SCS revisions. METHODS The clinical and radiological information of 141 patients with intractable chronic pain who underwent SCS implantation within the past 20 years was retrospectively reviewed. Paddle lead SCSs were used in this series. Statisti- cal analysis was conducted using Kaplan-Meier curves and Cox proportional-hazards regression. RESULTS Among 141 cases, 90 (64%) did not require any revision after SCS implantations. Removal of the SCS was required in 14 patients. The average pain score was significantly reduced (preimplantation score of 8 vs postimplantation score of 1.38; p < 0.0001). Younger age, male sex, obesity, a preimplantation pain score ≥ 8, and the presence of neuro- muscular pain were identified as predictors of the overall requirement for SCS revision. However, only a preimplantation pain score ≥ 8 was identified as a predictor of early failure of the SCS. COncLUSIONS Implantation of a paddle lead SCS is a relatively less invasive, safe, and effective procedure for pa- tients with intractable back pain.