Quantum-Gas Microscope for Fermionic Atoms
Total Page:16
File Type:pdf, Size:1020Kb
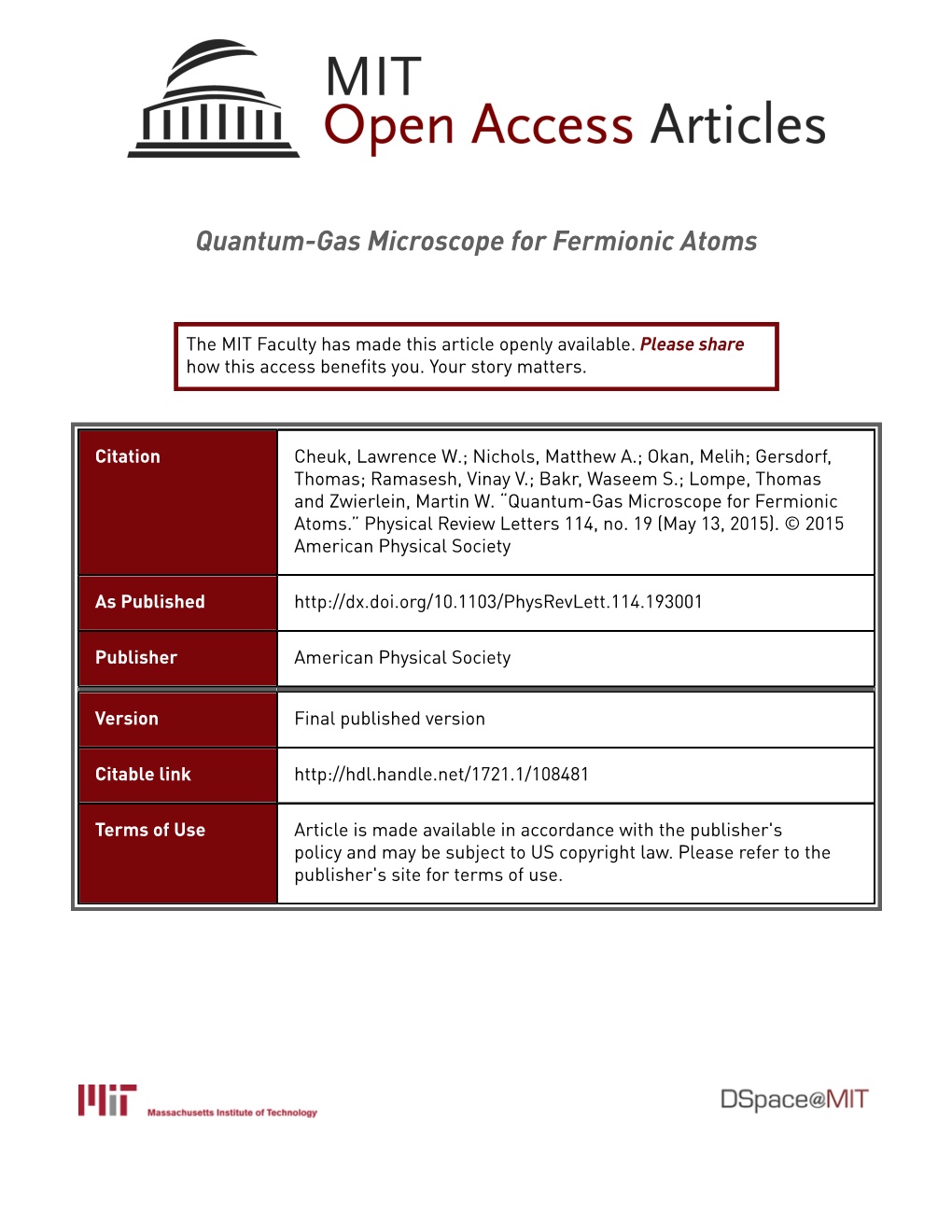
Load more
Recommended publications
-
Sideband Cooling
1 Laser collimation of a continuous beam of cold atoms using Zeeman-shift degenerate-Raman- sideband cooling G. Di Domenico,* N. Castagna, G. Mileti, and P. Thomann Observatoire cantonal, rue de l’Observatoire 58, 2000 Neuchâtel, Switzerland A. V. Taichenachev and V. I. Yudin Novosibirsk State University, Pirogova 2, Novosibirsk 630090, Russia Institute of Laser Physics SB RAS, Lavrent’eva 13/3, Novosibirsk 630090, Russia In this article we report on the use of degenerate-Raman-sideband cooling for the collimation of a continu- ous beam of cold cesium atoms in a fountain geometry. Thanks to this powerful cooling technique we have reduced the atomic beam transverse temperature from 60 K to 1.6 K in a few milliseconds. The longitu- dinal temperature of 80 K is not modified. The flux density, measured after a parabolic flight of 0.57 s, has been increased by a factor of 4 to approximately 107 at. s−1 cm−2 and we have identified a Sisyphus-like precooling mechanism which should make it possible to increase this flux density by an order of magnitude. ͉ ͘ I. INTRODUCTION cycle consists of two Raman transitions F=3, mF =3, n !͉3, 2, n−1͘!͉3, 1, n−2͘ followed by an optical pump- Since the discovery of laser cooling 1 , beams of slow [ ] ing cycle towards ͉3, 3, n−2͘. Each Raman transition re- and cold atoms have played an ever more important role in moves one vibrational quantum but the optical pumping con- high-precision experiments—e.g., in atomic interferometry serves n with high probability because the atoms are in the experiments 2,3 and atomic fountain clocks 4 . -
Subrecoil Raman Cooling of Cesium Atoms
EUROPHYSICS LETTERS 1 December 1994 Europhps. Lett., 28 (7), pp. 477-482 (1994) Subrecoil Raman Cooling of Cesium Atoms. J. REICHEL,0. MORICE,G. M. TINO(*)and C. SALOMON Laboratoire Kastkr Brossel, Ecok Normale Supdrieure 24 rue Lhomoncl, F-75251 Paris Cedes 05, France (received 3 August 1994; accepted in final form 18 October 1994) PACS. 32.80P - Optical cooling of atoms; trapping. PACS. 42.60 - Quantum optics. Abstract. - We use velocity-selective Raman pulses generated by two diode lasers to cool cesium atoms in 1 dimension to an r.m.8. of 1.2mm/s. This is about 1/3 of the single-photon recoil velocity v,= hk/M, where hk is the photon momentum and M the atom’s mass. The corresponding effective temperature is a factor of 9 below the single-photon recoil temperature given by kB T, /2 = 1/2Mv&. Because of the high cesium mass, this temperature is only (23 f 5) nanokelvin, the lowest 1D kinetic temperature reported to date. In recent years the temperature of laser-cooled neutral atoms has rapidly dropped[ll. Today there are two experimentally tested laser cooling methods that lead to atomic samples where the velocity spread is smaller than the atom’s recoil velocity after emission of a photon: velocity-selective coherent population trapping (VSCPT) [2,3] and Raman cooling [41. These cooling mechanisms were initially demonstrated in one dimension on He and Na, respectively, and they have recently been extended to higher dimensions [5,6]. They have in common the idea of letting the atoms randomly scatter photons until they fall by spontaneous emission into a velocity-selective state which is no longer coupled to the laser field. -
Chapter 2 Cooling to the Ground State of Axial Motion
11 Chapter 2 Cooling to the ground state of axial motion 2.1 In situ cooling: motivation and background While measurements in Ref. [13] established a lifetime of 2–3 seconds for atoms trapped in the FORT, these were atoms “in the dark,” i.e., only interrogated once after a variable time t to determine if they were still present. Subsequent experiments have required the trapped atom to interact repeatedly with fields applied either along the cavity axis or from the side of the cavity. In this case, lifetimes have in practice been limited to hundreds of milliseconds due to heating of the atom by the applied fields. In order to counterbalance these heating processes, one can imagine some method for cooling the atom in situ after it has been loaded into the trap: cooling intervals could then be interleaved as often as necessary between experimental cycles. Inter- leaved cooling would allow more cycles to occur before the atom was eventually heated out of the trap; ideally, the interrogation time would be limited only by the intrinsic FORT lifetime. In addition, cooling would localize the atom’s center-of-mass motion within a single FORT well. As the atom-cavity coupling g is spatially dependent, an atom moving within a potential well sees a periodically modulated coupling whose amplitude is proportional to temperature. Effective cooling would thus restrict the range of g values that the atom could sample. 12 One means of cooling a trapped atom is by driving a series of Raman transitions that successively lower the atom’s vibrational quantum number n, a process known as sideband cooling. -
Arxiv:1909.08894V1 [Physics.Atom-Ph] 19 Sep 2019 on Degenerate Raman Sideband Cooling (Drsc), Which Was Originally Developed for the Loss-Free Cooling of Neu- III
Ground-State Cooling of a Single Atom in a High-Bandwidth Cavity Eduardo Uru~nuela,∗ Wolfgang Alt, Elvira Keiler, Dieter Meschede, Deepak Pandey, Hannes Pfeifer, and Tobias Macha Institut f¨urAngewandte Physik, Universit¨atBonn, Wegelerstraße 8, 53115 Bonn, Germany We report on vibrational ground-state cooling of a single neutral atom coupled to a high- bandwidth Fabry-P´erotcavity. The cooling process relies on degenerate Raman sideband tran- sitions driven by dipole trap beams, which confine the atoms in three dimensions. We infer a one-dimensional motional ground state population close to 90 % by means of Raman spectroscopy. Moreover, lifetime measurements of a cavity-coupled atom exceeding 40 s imply three-dimensional cooling of the atomic motion, which makes this resource-efficient technique particularly interesting for cavity experiments with limited optical access. I. INTRODUCTION dimensional (1D) ground state population close to 90 %. Single atoms coupled to optical resonators are one of the most fundamental platforms in quantum optics and II. EXPERIMENTAL SETUP find applications in many tasks of quantum information science [1{5]. As a light-matter interface, they are a Our setup consists of a single 87Rb atom trapped at promising building block for long-distance quantum com- the center of a high-bandwidth FFPC [16] with CQED munication [6,7] due to their ability to provide single parameters (g; κ, γ) = 2π · (80; 41; 3) MHz, where g is the photons of controlled shape [2] and to store quantum in- single atom-cavity coupling strength. One of the fiber formation [8]. Ultimately, communication will always be mirrors has a higher transmission, providing a single- pushed towards high rates, such that information carry- sided cavity with a highly directional input-output chan- ing photons and corresponding resonators need to have nel [17]. -
Towards Resolved-Sideband Raman Cooling of a Single Rb-87 Atom in A
TOWARDS RESOLVED-SIDEBAND RAMAN COOLING OF A SINGLE 87Rb ATOM IN A FORT LEE JIANWEI NATIONAL UNIVERSITY OF SINGAPORE 2010 TOWARDS RESOLVED-SIDEBAND RAMAN COOLING OF A SINGLE 87Rb ATOM IN A FORT LEE JIANWEI B. Phy. (Hons.), NUS A THESIS SUBMITTED FOR THE DEGREE OF MASTER OF SCIENCE DEPARTMENT OF PHYSICS NATIONAL UNIVERSITY OF SINGAPORE SINGAPORE 2010 i i Acknowledgements First thanks goes foremost to my parents, who are the wind beneath my wings, whose love language is to feed and maintain me everyday without complaining, and being willing to travel all the way to Changi to get me a laptop dc power supply, despite the fact that I spend most of my time out of home. Special thanks goes to Christian Kurtsiefer, my research advisor, who proofread the thesis drafts, taught me quantum optics, electronics and ex- posing me to conferences that I didn’t think I fully deserved to go. Thanks to Gleb Maslennikov, before whom I’ve never met a real-life Rus- sian but now I find them very amusing. He’s taught me about cavities, milling, lathing, girls, chocolates and vodka; a competent experimental physi- cist and a patient mentor. Thanks to Syed Abdullah Aljunid who has been indispensible to the quan- tum optics team. His proficiency in almost every experimental equipment and technique, from atomic physics to optics to hardware to programming to troubleshooting to turning knobs near the edge of human sensitivity, has been a great testimony to what patience and common sense can do. Thanks for staying up as long as I have for the experiments and also giving me free rides home. -
Sub-Doppler Laser Cooling of 39K Via the 4S→5P Transition
SciPost Phys. 6, 047 (2019) Sub-Doppler laser cooling of 39K via the 4S 5P transition ! Govind Unnikrishnan?, Michael Gröbner and Hanns-Christoph Nägerl Institut für Experimentalphysik und Zentrum für Quantenphysik, Universität Innsbruck, 6020 Innsbruck, Austria ? [email protected] Abstract We demonstrate sub-Doppler laser cooling of 39K using degenerate Raman sideband cool- ing via the 4S1=2 5P1=2 transition at 404.8 nm. By using an optical lattice in combina- tion with a magnetic! field and optical pumping beams, we obtain a spin-polarized sample of up to 5.6 107 atoms cooled down to a sub-Doppler temperature of 4 µK, reaching × 9 3 5 a peak density of 3.9 10 atoms/cm , a phase-space density greater than 10− , and an average vibrational level× of ν = 0.6 in the lattice. This work opens up the possibility of implementing a single-siteh imagingi scheme in a far-detuned optical lattice utilizing shorter wavelength transitions in alkali atoms, thus allowing improved spatial resolu- tion. Copyright G. Unnikrishnan et al. Received 07-11-2018 This work is licensed under the Creative Commons Accepted 29-03-2019 Check for Attribution 4.0 International License. Published 18-04-2019 updates Published by the SciPost Foundation. doi:10.21468/SciPostPhys.6.4.047 Contents 1 Introduction1 2 Background 2 2.1 The 4S 5P transition2 2.2 Degenerate! Raman sideband cooling5 3 Experiment 5 3.1 Setup 5 3.2 Measurements7 4 Conclusions 12 5 Acknowledgements 13 References 13 1 SciPost Phys. 6, 047 (2019) 1 Introduction Sub-Doppler laser cooling is an important intermediate step between Doppler cooling [1–3] and evaporative cooling in most quantum gas experiments. -
Direct Laser Cooling to Bose-Einstein Condensation in a Dipole Trap
PHYSICAL REVIEW LETTERS 122, 203202 (2019) Editors' Suggestion Direct Laser Cooling to Bose-Einstein Condensation in a Dipole Trap † Alban Urvoy,* Zachary Vendeiro,* Joshua Ramette, Albert Adiyatullin, and Vladan Vuletić Department of Physics, MIT-Harvard Center for Ultracold Atoms and Research Laboratory of Electronics, Massachusetts Institute of Technology, Cambridge, Massachusetts 02139, USA (Received 27 February 2019; published 24 May 2019) We present a method for producing three-dimensional Bose-Einstein condensates using only laser cooling. The phase transition to condensation is crossed with 2.5 × 10487Rb atoms at a temperature of Tc ¼ 0.6 μK after 1.4 s of cooling. Atoms are trapped in a crossed optical dipole trap and cooled using Raman cooling with far-off-resonant optical pumping light to reduce atom loss and heating. The achieved temperatures are well below the effective recoil temperature. We find that during the final cooling stage at atomic densities above 1014 cm−3, careful tuning of trap depth and optical-pumping rate is necessary to evade heating and loss mechanisms. The method may enable the fast production of quantum degenerate gases in a variety of systems including fermions. DOI: 10.1103/PhysRevLett.122.203202 Quantum degenerate gases provide a flexible platform In this Letter, we demonstrate Raman cooling [9,18–21] with applications ranging from quantum simulations of of an ensemble of 87Rb atoms into the quantum degenerate many-body interacting systems [1] to precision measure- regime, without evaporative cooling. Starting with up to ments [2]. The standard technique for achieving quantum 1 × 105 atoms in an optical dipole trap, the transition to degeneracy is laser cooling followed by evaporative cool- BEC is reached with up to 2.5 × 104 atoms within a ing [3] in magnetic [4–6] or optical traps [7]. -
Resolved-Sideband Raman Cooling of a Bound Atom to the 3D Zero-Point Energy
VOLUME 75, NUMBER 22 PH YS ICAL REVIEW LETTERS 27 NovEMBER 1995 Resolved-Sideband Raman Cooling of a Bound Atom to the 3D Zero-Point Energy C. Monroe, D. M. Meekhof, B.E. King, S.R. Jefferts, W. M. Itano, and D. J. Wineland Time and Frequency Division, National Institute of Standards and Technology, Boulder, Colorado 80303 P. Gould Department of Physics, University of Connecticut, Storrs, Connecticut 06269 (Received 19 December 1994) We report laser cooling of a single 9Be ion held in a rf (Paul) ion trap to where it occupies the quantum-mechanical ground state of motion. With the use of resolved-sideband stimulated Raman cooling, the zero point of motion is achieved 98% of the time in 1D and 92% of the time in 3D. Cooling to the zero-point energy appears to be a crucial prerequisite for future experiments such as the realization of simple quantum logic gates applicable to quantum computation. PACS numbers: 32.80.Pj, 42.50.Vk, 42.65.Dr Dramatic progress in the field of atomic laser cooling We cool a single beryllium ion bound in a rf (Paul) ion has provided cooling of free or weakly bound atoms trap to near the zero-point energy using resolved-sideband to temperatures near the Doppler cooling limit [1], near laser cooling with stimulated Raman transitions along the photon recoil limit [2], and, more recently, below the lines suggested in Ref. [6]. The idea of resolved- the photon recoil limit [3,4]. For a tightly bound atom, sideband laser cooling with a single-photon transition is as a more natural energy scale is given by the quantized follows [12]. -
Raman Sideband Cooling and Coherent Manipulation of Trapped Ions
Raman Sideband Cooling and Coherent Manipulation of Trapped Ions A thesis submitted for the degree of Doctor of Philosophy 0.7 0.6 0.5 Fraction of ions shelved 0.4 0.3 0.2 −1000 −800 −600 −400 −200 0 200 400 600 800 1000 Raman detuning /kHz Simon Webster Hilary Term St. John’s College 2005 Oxford Abstract This thesis describes experiments primarily concerned with the manipulation of the spin and quantum motional state of single trapped ions and pairs of ions. A two stage photoionization system is described. This allows the loading of ions of a specific isotope of calcium into our trap. We measure the absolute cross section for 1 photoionization from the 4s4p P1 state of neutral Ca. We demonstrate the loading and cooling of all the naturally occurring isotopes of calcium, including of pure crystals of 43Ca+. A magnetic resonance is used to determine accurately the Zeeman splitting of the ground state of a 40Ca+ ion. The coherent manipulation of the spin state of a 40Ca+ ion using this magnetic resonance is shown. We observe high quality Rabi oscillations between the two spin states with π times of order 100 µs and with decay times of order milliseconds. By using multiple pulses of this magnetic resonance in a Ramsey type experiment we are able to measure the net light shift induced on the two spin states by a circularly polarised far-off-resonant beam, and thus deduce its intensity precisely, and also ensure that such a beam is linearly polarised to high accuracy. We demonstrate a laser-driven rotation of the qubit with a π time of order a few microseconds. -
Raman Cooling of Solids Through Photonic Density of States Engineering
Raman Cooling of Solids through Photonic Density of States Engineering Yin-Chung Chen1, Gaurav Bahl1∗ 1Mechanical Science and Engineering, University of Illinois at Urbana-Champaign, Urbana, Illinois, USA ∗To whom correspondence should be addressed; E-mail: [email protected]. Abstract The laser cooling of vibrational states of solids has been achieved through photoluminescence in rare-earth elements, optical forces in optomechanics, and the Brillouin scattering light-sound interaction. The net cooling of solids through spontaneous Raman scattering, and laser refrigeration of indirect band gap semiconductors, both remain unsolved challenges. Here, we analytically show that photonic den- sity of states (DoS) engineering can address the two fundamental re- quirements for achieving spontaneous Raman cooling: suppressing the dominance of Stokes (heating) transitions, and the enhancement of anti-Stokes (cooling) efficiency beyond the natural optical absorption of the material. We develop a general model for the DoS modifica- tion to spontaneous Raman scattering probabilities, and elucidate the necessary and minimum condition required for achieving net Raman cooling. With a suitably engineered DoS, we establish the enticing possibility of refrigeration of intrinsic silicon by annihilating phonons from all its Raman-active modes simultaneously, through a single tele- com wavelength pump. This result points to a highly flexible approach for laser cooling of any transparent semiconductor, including indirect band gap semiconductors, far away from significant optical absorption, band-edge states, excitons, or atomic resonances. arXiv:1507.08936v1 [physics.optics] 31 Jul 2015 1 Introduction The photon-induced annihilation of thermal quanta from matter, i.e. laser cooling, is of key importance in ultracold science and has been instrumental in the creation of gas phase quantum condensates, matter-wave interfer- ometry, and macroscopic tests of quantum mechanics. -
Optimization of Anisotropic Photonic Density of States for Raman Cooling of Solids
PHYSICAL REVIEW A 97, 043835 (2018) Optimization of anisotropic photonic density of states for Raman cooling of solids Yin-Chung Chen,1 Indronil Ghosh,1 André Schleife,2 P. Scott Carney,3 and Gaurav Bahl1,* 1Department of Mechanical Science and Engineering, University of Illinois at Urbana-Champaign, Urbana, Illinois 61801, USA 2Department of Materials Science and Engineering, University of Illinois at Urbana-Champaign, Urbana, Illinois 61801, USA 3The Institute of Optics, University of Rochester, Rochester, New York 14627, USA (Received 23 January 2018; published 16 April 2018) Optical refrigeration of solids holds tremendous promise for applications in thermal management. It can be achieved through multiple mechanisms including inelastic anti-Stokes Brillouin and Raman scattering. However, engineering of these mechanisms remains relatively unexplored. The major challenge lies in the natural unfavorable imbalance in transition rates for Stokes and anti-Stokes scattering. We consider the influence of anisotropic photonic density of states on Raman scattering and derive expressions for cooling in such photonically anisotropic systems. We demonstrate optimization of the Raman cooling figure of merit considering all possible orientations for the material crystal and two example photonic crystals. We find that the anisotropic description of the photonic density of states and the optimization process is necessary to obtain the best Raman cooling efficiency for systems having lower symmetry. This general result applies to a wide array of other -
Raman Sideband Cooling of a 138Ba Ion Using a Zeeman Interval
PHYSICAL REVIEW A 93, 053415 (2016) Raman sideband cooling of a 138Ba+ ion using a Zeeman interval Christopher M. Seck,1 Mark G. Kokish,1 Matthew R. Dietrich,1,2 and Brian C. Odom1,* 1Department of Physics and Astronomy, Northwestern University, Evanston, Illinois 60208, USA 2Physics Division, Argonne National Laboratory, Argonne, Illinois 60439, USA (Received 31 March 2016; published 17 May 2016) Motional ground state cooling and internal state preparation are important elements for quantum logic spectroscopy (QLS), a class of quantum information processing. Since QLS does not require the high gate fidelities usually associated with quantum computation and quantum simulation, it is possible to make simplifying choices in ion species and quantum protocols at the expense of some fidelity. Here, we report sideband cooling and motional state detection protocols for 138Ba+ of sufficient fidelity for QLS without an extremely narrow-band 138 + laser or the use of a species with hyperfine structure. We use the two S1/2 Zeeman sublevels of Ba to Raman sideband cool a single ion to the motional ground state. Because of the small Zeeman splitting, continuous near-resonant Raman sideband cooling of 138Ba+ requires only the Doppler cooling lasers and two additional acousto-optic modulators. Observing the near-resonant Raman optical pumping fluorescence, we extract relevant experimental parameters and demonstrate a final average motional quantum number n¯ 1. We additionally employ a second, far-off-resonant laser driving Raman π pulses between the two Zeeman sublevels to provide motional state detection for QLS and to confirm the sideband cooling efficiency, measuring a final n¯ = 0.15(6) .