Locating Ice Sheet Grounding Lines Using Satellite Radar Interferometry and Altimetry
Total Page:16
File Type:pdf, Size:1020Kb
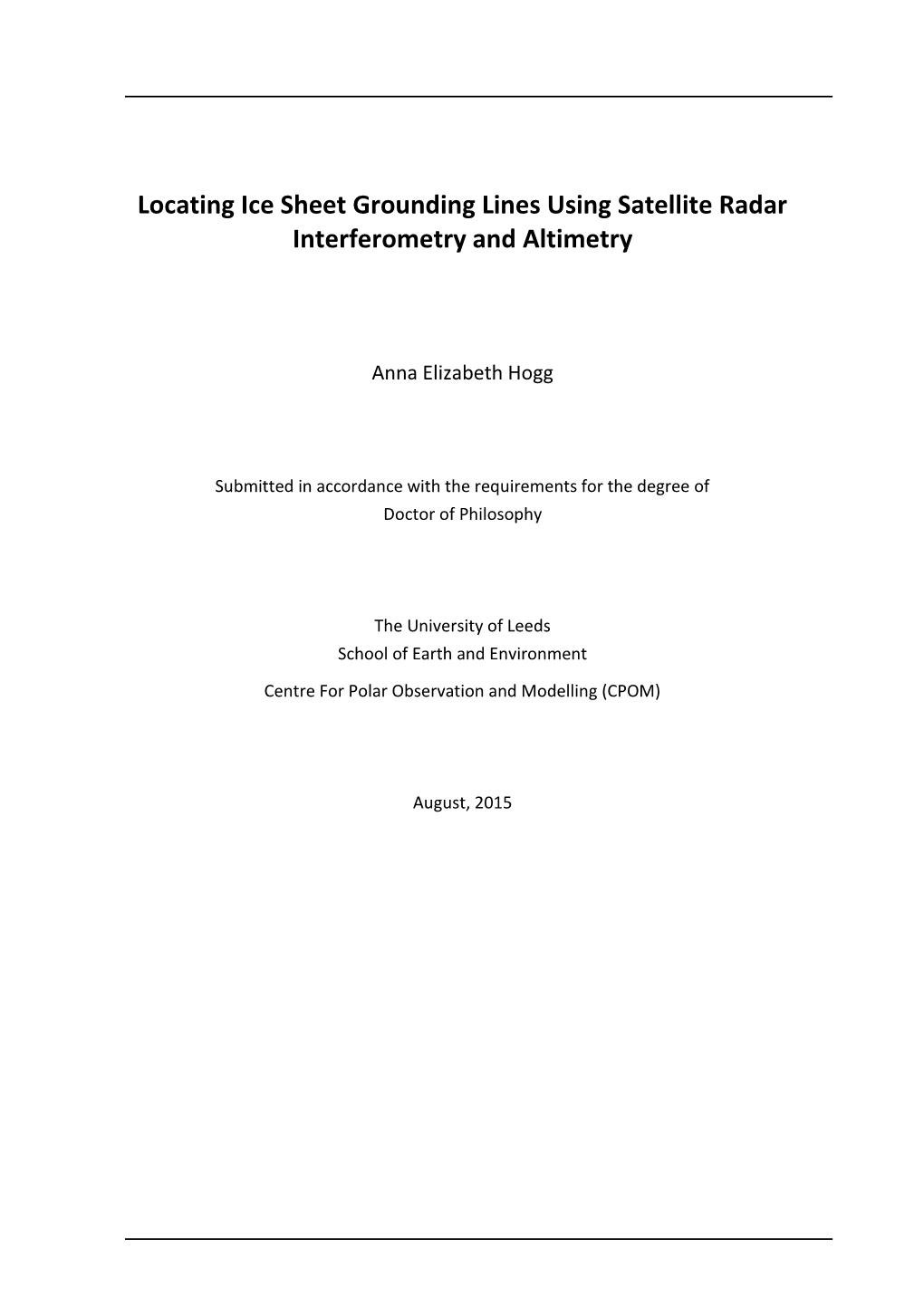
Load more
Recommended publications
-
OIB Antarctic Flight 10, Antarctica Peninsula #2 Aircraft DC-8 Flight
OIB Antarctic Flight 10, Antarctica Peninsula #2 Aircraft DC-8 Flight Number DC8-100119 Flt Req # 108002 Flight Hours 9.4 Date 10/31/09 Purpose of Flight ICE Bridge Peninsula-2 Aircraft Status Airworthy Sensor Status All installed sensors operational. Significant Issues None Accomplishments Low level survey over a variety of Peninsula targets. Cloud cover obscured about 400 km of the planned 2300 km lines (~18% loss). Cloud cover affected the regions south of 72 deg. Sensors worked throughout. LVIS collected approximately one hour of sea ice data during the transit. McCords, Snow and Ku Band radars were operational throughout the target areas. Made a low pass over Palmer Station and conducted two passes over Punta Arenas for instrument calibration. Planned events Down day tomorrow Flight Summary Peninsula-2, FLT 11 Saturday, October 31, 2009 Seelye Martin (Mission Principal Investigator): Weather Summary: this is the first potentially clear day on the Peninsula. AMPS forecast shows a major system moving from west, reaching peninsula about 1800 UTC. Peninsula south of about 73 S is under heavy clouds. From their Antarctic forecast network, Fight Services shows that the lower Peninsula has broken cloud decks at 1,500 and 3,000 ft. Quoting John Sonntag, “For any other area, we would not fly, but the peninsula is open so infrequently that we have to try it.” Suspect we will have cloud problems at both north and south end of flight lines. Mission Description: This is a repeat of ATM lines flown by the Chilean/NASA P-3 in October 2008. The lines cover the Fleming Glacier, Mobile Oil Inlet and a pair of ICESat lines, one on the George-V ice shelf and a parallel one over Palmer Land, plus a single pass down the Crane Glacier. -
Environmental Drivers of Circum-Antarctic Glacier and Ice Shelf Front Retreat Over the Last Two Decades Celia A
Environmental Drivers of Circum-Antarctic Glacier and Ice Shelf Front Retreat over the Last Two Decades Celia A. Baumhoer1, Andreas. J. Dietz1, Christof Kneisel2, Heiko Paeth2 and Claudia Kuenzer1,2 1German Remote Sensing Data Center (DFD), German Aerospace Center (DLR), Muenchner Strasse 20, D-82234 Wessling, 5 Germany 2Institute of Geography and Geology, University Wuerzburg, Am Hubland, D-97074 Wuerzburg, Germany Correspondence to: Celia A. Baumhoer ([email protected]) Abstract. The safety band of Antarctica, consisting of floating glacier tongues and ice shelves, buttresses ice discharge of the Antarctic Ice Sheet. Recent disintegration events of ice shelves along with glacier retreat indicate a weakening of this important 10 safety band. Predicting calving front retreat is a real challenge due to complex ice dynamics in a data-scarce environment that are unique for each ice shelf and glacier. We explore to what extent easy to access remote sensing and modelling data can help to define environmental conditions leading to calving front retreat. For the first time, we present a circum-Antarctic record of glacier and ice shelf front change over the last two decades in combination with environmental variables such as air temperature, sea ice days, snowmelt, sea surface temperature and wind direction. We find that the Antarctic Ice Sheet area 15 decreased by -29,618±1,193 km2 in extent between 1997-2008 and gained an area of 7,108±1,029km2 between 2009 and 2018. Retreat concentrated along the Antarctic Peninsula and West Antarctica including the biggest ice shelves (Ross and Ronne). In several cases, glacier and ice shelf retreat occurred in conjunction with one or several changes in environmental variables. -
Modelling Ice Dynamic Sea-Level Rise from the Antarctic Peninsula Ice Sheet
Modelling ice dynamic sea-level rise from the Antarctic Peninsula Ice Sheet Clemens Schannwell A thesis submitted to the University of Birmingham for the degree of DOCTOR OF PHILOSOPHY. School of Geography, Earth and Environmental Sciences College of Life and Environmental Sciences University of Birmingham May 2017 University of Birmingham Research Archive e-theses repository This unpublished thesis/dissertation is copyright of the author and/or third parties. The intellectual property rights of the author or third parties in respect of this work are as defined by The Copyright Designs and Patents Act 1988 or as modified by any successor legislation. Any use made of information contained in this thesis/dissertation must be in accordance with that legislation and must be properly acknowledged. Further distribution or reproduction in any format is prohibited without the permission of the copyright holder. Abstract The Antarctic Peninsula (AP) has been one of the most rapidly warming regions on this planet. This warming has been accompanied by major glaciological changes such as tidewater glacier retreat, ice-shelf retreat and collapse alongside acceleration of outlet glaciers in response to ice-shelf removal. As faster flowing glaciers deliver more ice from the ice sheet’s interior to the margins, the AP has been identified as an important contributor to global sea-level rise (SLR). However, comprehensive SLR projections of the AP induced by ice dynamics over the next three centuries are still lacking. The period to 2300 is selected as there are high quality climate forcing data available. This timeframe is also of particular interest for policy makers. -
I!Ij 1)11 U.S
u... I C) C) co 1 USGS 0.. science for a changing world co :::2: Prepared in cooperation with the Scott Polar Research Institute, University of Cambridge, United Kingdom Coastal-change and glaciological map of the (I) ::E Bakutis Coast area, Antarctica: 1972-2002 ;::+' ::::r ::J c:r OJ ::J By Charles Swithinbank, RichardS. Williams, Jr. , Jane G. Ferrigno, OJ"" ::J 0.. Kevin M. Foley, and Christine E. Rosanova a :;:,..... CD ~ (I) I ("') a Geologic Investigations Series Map I- 2600- F (2d ed.) OJ ~ OJ '!; :;:, OJ ::J <0 co OJ ::J a_ <0 OJ n c; · a <0 n OJ 3 OJ "'C S, ..... :;:, CD a:r OJ ""a. (I) ("') a OJ .....(I) OJ <n OJ n OJ co .....,...... ~ C) .....,0 ~ b 0 C) b C) C) T....., Landsat Multispectral Scanner (MSS) image of Ma rtin and Bea r Peninsulas and Dotson Ice Shelf, Bakutis Coast, CT> C) An tarctica. Path 6, Row 11 3, acquired 30 December 1972. ? "T1 'N 0.. co 0.. 2003 ISBN 0-607-94827-2 U.S. Department of the Interior 0 Printed on rec ycl ed paper U.S. Geological Survey 9 11~ !1~~~,11~1!1! I!IJ 1)11 U.S. DEPARTMENT OF THE INTERIOR TO ACCOMPANY MAP I-2600-F (2d ed.) U.S. GEOLOGICAL SURVEY COASTAL-CHANGE AND GLACIOLOGICAL MAP OF THE BAKUTIS COAST AREA, ANTARCTICA: 1972-2002 . By Charles Swithinbank, 1 RichardS. Williams, Jr.,2 Jane G. Ferrigno,3 Kevin M. Foley, 3 and Christine E. Rosanova4 INTRODUCTION areas Landsat 7 Enhanced Thematic Mapper Plus (ETM+)), RADARSAT images, and other data where available, to compare Background changes over a 20- to 25- or 30-year time interval (or longer Changes in the area and volume of polar ice sheets are intri where data were available, as in the Antarctic Peninsula). -
First Exposure Ages from the Amundsen Sea Embayment, West Antarctica: the Late Quaternary Context for Recent Thinning of Pine Island, Smith, and Pope Glaciers
View metadata, citation and similar papers at core.ac.uk brought to you by CORE provided by Electronic Publication Information Center First exposure ages from the Amundsen Sea Embayment, West Antarctica: The Late Quaternary context for recent thinning of Pine Island, Smith, and Pope Glaciers Joanne S. Johnson British Antarctic Survey, High Cross, Madingley Road, Cambridge CB3 0ET, UK Michael J. Bentley Department of Geography, Durham University, South Road, Durham DH1 3LE, UK and British Antarctic Survey, High Cross, Madingley Road, Cambridge CB3 0ET, UK Karsten Gohl Alfred-Wegener-Institut für Polar- und Meeresforschung, Postfach 120161, D-27515 Bremerhaven, Germany ABSTRACT Dramatic changes (acceleration, thinning, and grounding-line retreat of major ice streams) in the Amundsen Sea sector of the West Antarctic Ice Sheet (WAIS) have been observed dur- ing the past two decades, but the millennial-scale context for these changes is not yet known. We present the fi rst surface exposure ages recording thinning of Pine Island, Smith, and Pope Glaciers, which all drain into the Amundsen Sea. From these we infer progressive thinning of Pine Island Glacier at an average rate of 3.8 ± 0.3 cm yr–1 for at least the past 4.7 k.y., and of Smith and Pope Glaciers at 2.3 ± 0.2 cm yr–1 over the past 14.5 k.y. These rates are more than an order of magnitude lower than the ~1.6 m yr–1 recorded by satellite altimetry for Pine Island Glacier in the period 1992–1996. Similarly low long-term rates (2.5–9 cm yr–1 since 10 ka) have been reported farther west in the Ford Ranges, Marie Byrd Land, but in that area, the same rates of thinning continue to the present day. -
Glacial Retreat in the Amundsen Sea Sector, West Antarctica E first Cosmogenic Evidence from Central Pine Island Bay and the Kohler Range
Quaternary Science Reviews 98 (2014) 166e173 Contents lists available at ScienceDirect Quaternary Science Reviews journal homepage: www.elsevier.com/locate/quascirev Glacial retreat in the Amundsen Sea sector, West Antarctica e first cosmogenic evidence from central Pine Island Bay and the Kohler Range * Julia Lindow a, , Marion Castex a, Hella Wittmann b, Joanne S. Johnson c, Frank Lisker a, Karsten Gohl d, Cornelia Spiegel a a Department of Geosciences, University of Bremen, Bremen, Germany b Helmholtz-Centre Potsdam e German Research Centre for Geosciences (GFZ), Potsdam, Germany c British Antarctic Survey, Natural Environment Research Council, High Cross, Madingley Road, Cambridge CB3 0ET, UK d Alfred Wegener Institute, Helmholtz-Centre for Polar and Marine Research (AWI), Bremerhaven, Germany article info abstract Article history: The Amundsen Sea Embayment of West Antarctica hosts one of the most rapidly changing sectors of the Received 14 February 2014 West Antarctic Ice Sheet. With the fastest-flowing ice streams in Antarctica, the region around Pine Is- Received in revised form land Bay is characterized by rapid ice-sheet thinning and grounding-line retreat. Published surface- 13 May 2014 exposure data are limited to a few isolated nunataks making it difficult to assess the long-term degla- Accepted 15 May 2014 cial history of the area. To address this, we correlate existing records of lateral ice-stream retreat from Available online 4 July 2014 marine sediment cores with onshore glacial thinning in two key areas of eastern Marie Byrd Land: the Kohler Range and Pine Island Bay. Our 10Be surface-exposure ages are the first from the isolated Kohler Keywords: Surface exposure dating Range and show that the nunataks there became ice-free between 8.6 and 12.6 ka. -
The Last Glaciation of Bear Peninsula, Central Amundsen Sea
Quaternary Science Reviews 178 (2017) 77e88 Contents lists available at ScienceDirect Quaternary Science Reviews journal homepage: www.elsevier.com/locate/quascirev The last glaciation of Bear Peninsula, central Amundsen Sea Embayment of Antarctica: Constraints on timing and duration revealed by in situ cosmogenic 14Cand10Be dating * Joanne S. Johnson a, , James A. Smith a, Joerg M. Schaefer b, Nicolas E. Young b, Brent M. Goehring c, Claus-Dieter Hillenbrand a, Jennifer L. Lamp b, Robert C. Finkel d, Karsten Gohl e a British Antarctic Survey, High Cross, Madingley Road, Cambridge CB3 0ET, UK b Lamont-Doherty Earth Observatory, Columbia University, Route 9W, Palisades, New York NY 10964, USA c Department of Earth & Environmental Sciences, Tulane University, New Orleans, LA 70118, USA d Lawrence Livermore National Laboratory, Center for Accelerator Mass Spectrometry, 7000 East Avenue Avenue, Livermore, CA 94550-9234, USA e Alfred Wegener Institute for Polar and Marine Research, Postfach 120161, D-27515 Bremerhaven, Germany article info abstract Article history: Ice streams in the Pine Island-Thwaites region of West Antarctica currently dominate contributions to sea Received 6 April 2017 level rise from the Antarctic ice sheet. Predictions of future ice-mass loss from this area rely on physical Received in revised form models that are validated with geological constraints on past extent, thickness and timing of ice cover. 18 October 2017 However, terrestrial records of ice sheet history from the region remain sparse, resulting in significant Accepted 1 November 2017 model uncertainties. We report glacial-geological evidence for the duration and timing of the last glaciation of Hunt Bluff, in the central Amundsen Sea Embayment. -
Grounding Line Retreat of Pope, Smith, and Kohler Glaciers, West
Geophysical Research Letters RESEARCH LETTER Grounding line retreat of Pope, Smith, and Kohler Glaciers, 10.1002/2016GL069287 West Antarctica, measured with Sentinel-1a radar Key Points: interferometry data • Sentinel-1 is a breakthrough mission for systematic, widespread, 1 1 1,2 1 2 continued, grounding line mapping B. Scheuchl , J. Mouginot , E. Rignot , M. Morlighem , and A. Khazendar in Antarctica • Pope, Smith, and Kohler Glaciers 1Department of Earth System Science, University of California, Irvine, CA, USA, 2Jet Propulsion Laboratory, California are undergoing major changes. Institute of Technology, Pasadena, California, USA The Grounding line of Smith Glacier continues to retreat at 2 km/yr • Crosson and Dotson Ice Shelves lost many pinning points, which Abstract We employ Sentinel-1a C band satellite radar interferometry data in Terrain Observation with significantly threatens their stability Progressive Scans mode to map the grounding line and ice velocity of Pope, Smith, and Kohler glaciers, in in years to come West Antarctica, for the years 2014–2016 and compare the results with those obtained using Earth Remote Sensing Satellites (ERS-1/2) in 1992, 1996, and 2011. We observe an ongoing, rapid grounding line retreat Supporting Information: of Smith at 2 km/yr (40 km since 1996), an 11 km retreat of Pope (0.5 km/yr), and a 2 km readvance of • Supporting Information S1 Kohler since 2011. The variability in glacier retreat is consistent with the distribution of basal slopes, i.e., fast along retrograde beds and slow along prograde beds. We find that several pinning points holding Correspondence to: Dotson and Crosson ice shelves disappeared since 1996 due to ice shelf thinning, which signal the ongoing B. -
Major Increase in West Antarctic Glacial Loss
Antarctic Science News posted on 27/3/2014 compiled by Dr. Alvarinho J. Luis Major increase in West Antarctic glacial loss Six massive glaciers in West Antarctica are moving faster than they did 40 years ago, causing more ice to discharge into the ocean and global sea level to rise, according to new research. The amount of ice draining collectively from those half-dozen glaciers increased by 77 % from 1973 to 2013. Pine Island Glacier, the most active of the studied glaciers, has accelerated by 75% in 40 years, according to the research published in GRL. Thwaites Glacier, the widest glacier, started to accelerate in 2006, following a decade of stability. The study is the first to look at the ice coming off the six most active West Antarctic glaciers over such an extended time period. Almost 10 % of the world's sea-level rise per year comes from just these six glaciers. The researchers found was a sustained increase in ice discharge -- which has a significant impact on sea level rise. The researchers studied the Pine Island, Thwaites, Haynes, Smith, Pope and Kohler glaciers, all of which discharge ice into a vast bay known as the Amundsen Sea Embayment in West Antarctica. The amount of ice released by these six glaciers each year is comparable to the amount of ice draining from the entire Greenland Ice Sheet annually, Mouginot said. If melted completely, the glaciers' disappearance would raise sea levels another 1.2 meters , according to co-author and UC-Irvine Professor Eric Rignot. The decades of increasing speeds and ice loss are "a strong indication of a major, long-term leakage of ice into the ocean from that sector of Antarctica," noted Rignot. -
Coastal-Change and Glaciological Map of the Larsen Ice Shelf Area, Antarctica: 1940–2005
Prepared in cooperation with the British Antarctic Survey, the Scott Polar Research Institute, and the Bundesamt für Kartographie und Geodäsie Coastal-Change and Glaciological Map of the Larsen Ice Shelf Area, Antarctica: 1940–2005 By Jane G. Ferrigno, Alison J. Cook, Amy M. Mathie, Richard S. Williams, Jr., Charles Swithinbank, Kevin M. Foley, Adrian J. Fox, Janet W. Thomson, and Jörn Sievers Pamphlet to accompany Geologic Investigations Series Map I–2600–B 2008 U.S. Department of the Interior U.S. Geological Survey U.S. Department of the Interior DIRK KEMPTHORNE, Secretary U.S. Geological Survey Mark D. Myers, Director U.S. Geological Survey, Reston, Virginia: 2008 For product and ordering information: World Wide Web: http://www.usgs.gov/pubprod Telephone: 1-888-ASK-USGS For more information on the USGS--the Federal source for science about the Earth, its natural and living resources, natural hazards, and the environment: World Wide Web: http://www.usgs.gov Telephone: 1-888-ASK-USGS Any use of trade, product, or firm names is for descriptive purposes only and does not imply endorsement by the U.S. Government. Although this report is in the public domain, permission must be secured from the individual copyright owners to reproduce any copyrighted materials contained within this report. Suggested citation: Ferrigno, J.G., Cook, A.J., Mathie, A.M., Williams, R.S., Jr., Swithinbank, Charles, Foley, K.M., Fox, A.J., Thomson, J.W., and Sievers, Jörn, 2008, Coastal-change and glaciological map of the Larsen Ice Shelf area, Antarctica: 1940– 2005: U.S. Geological Survey Geologic Investigations Series Map I–2600–B, 1 map sheet, 28-p. -
Sustained Increase in Ice Discharge from the Amundsen Sea 10.1002/2013GL059069 Embayment, West Antarctica, from 1973 to 2013
UC San Diego UC San Diego Previously Published Works Title Dissipative selection of low-frequency modes in a reduced-gravity basin Permalink https://escholarship.org/uc/item/52c3s1t5 Journal Journal of Physical Oceanography, 31(1) ISSN 0022-3670 Authors Cessi, P Primeau, F Publication Date 2001 DOI 10.1175/1520-0485(2001)031<0127:DSOLFM>2.0.CO;2 License https://creativecommons.org/licenses/by/4.0/ 4.0 Peer reviewed eScholarship.org Powered by the California Digital Library University of California GeophysicalResearchLetters RESEARCH LETTER Sustained increase in ice discharge from the Amundsen Sea 10.1002/2013GL059069 Embayment, West Antarctica, from 1973 to 2013 Key Points: J. Mouginot1, E. Rignot1,2, and B. Scheuchl1 • Sustained ASE mass flux increase: 77% since 1973 1Department of Earth System Science, University of California, Irvine, California, USA, 2Jet Propulsion Laboratory, • Thwaites accelerated by 33% during California Institute of Technology, Pasadena, California, USA the last 6 years • Speed changes are pervasive and rapid: major implications for ice flow modeling Abstract We combine measurements of ice velocity from Landsat feature tracking and satellite radar interferometry, and ice thickness from existing compilations to document 41 years of mass flux from the Amundsen Sea Embayment (ASE) of West Antarctica. The total ice discharge has increased by 77% since Supporting Information: • Readme 1973. Half of the increase occurred between 2003 and 2009. Grounding-line ice speeds of Pine Island Glacier • Table S1 stabilized between 2009 and 2013, following a decade of rapid acceleration, but that acceleration reached • Table S2 far inland and occurred at a rate faster than predicted by advective processes. -
Basal Friction of Fleming Glacier, Antarctica – Part 1: Sensitivity of Inversion to Temperature and Bedrock Uncertainty
The Cryosphere, 12, 2637–2652, 2018 https://doi.org/10.5194/tc-12-2637-2018 © Author(s) 2018. This work is distributed under the Creative Commons Attribution 4.0 License. Basal friction of Fleming Glacier, Antarctica – Part 1: Sensitivity of inversion to temperature and bedrock uncertainty Chen Zhao1,3, Rupert M. Gladstone2, Roland C. Warner3, Matt A. King1, Thomas Zwinger4, and Mathieu Morlighem5 1School of Technology, Environments and Design, University of Tasmania, Hobart, Australia 2Arctic Centre, University of Lapland, Rovaniemi, Finland 3Antarctic Climate & Ecosystems Cooperative Research Centre, University of Tasmania, Hobart, Australia 4CSC – IT Center for Science Ltd., Espoo, Finland 5Department of Earth System Science, University of California, Irvine, CA 92697-3100, USA Correspondence: Chen Zhao ([email protected]) Received: 30 October 2017 – Discussion started: 2 January 2018 Revised: 17 July 2018 – Accepted: 20 July 2018 – Published: 15 August 2018 Abstract. Many glaciers in the Antarctic Peninsula are now ice discharge over recent decades, which has led to a signif- rapidly losing mass. Understanding of the dynamics of these icant contribution to global sea level rise (Cook et al., 2016; fast-flowing glaciers, and their potential future behaviour, Gardner et al., 2018; Wouters et al., 2015). Understanding the can be improved through ice sheet modelling studies. In- underlying processes is crucial to improve modelling of ice verse methods are commonly used in ice sheet models to dynamics and enable reliable predictions of contributions to infer the spatial distribution of a basal friction coefficient, sea level change, especially for fast-flowing outlet glaciers. which has a large effect on the basal velocity and ice de- The high velocities of fast-flowing outlet glaciers arise formation.