Mid-Infrared Properties of Local Active Galactic Nuclei
Total Page:16
File Type:pdf, Size:1020Kb
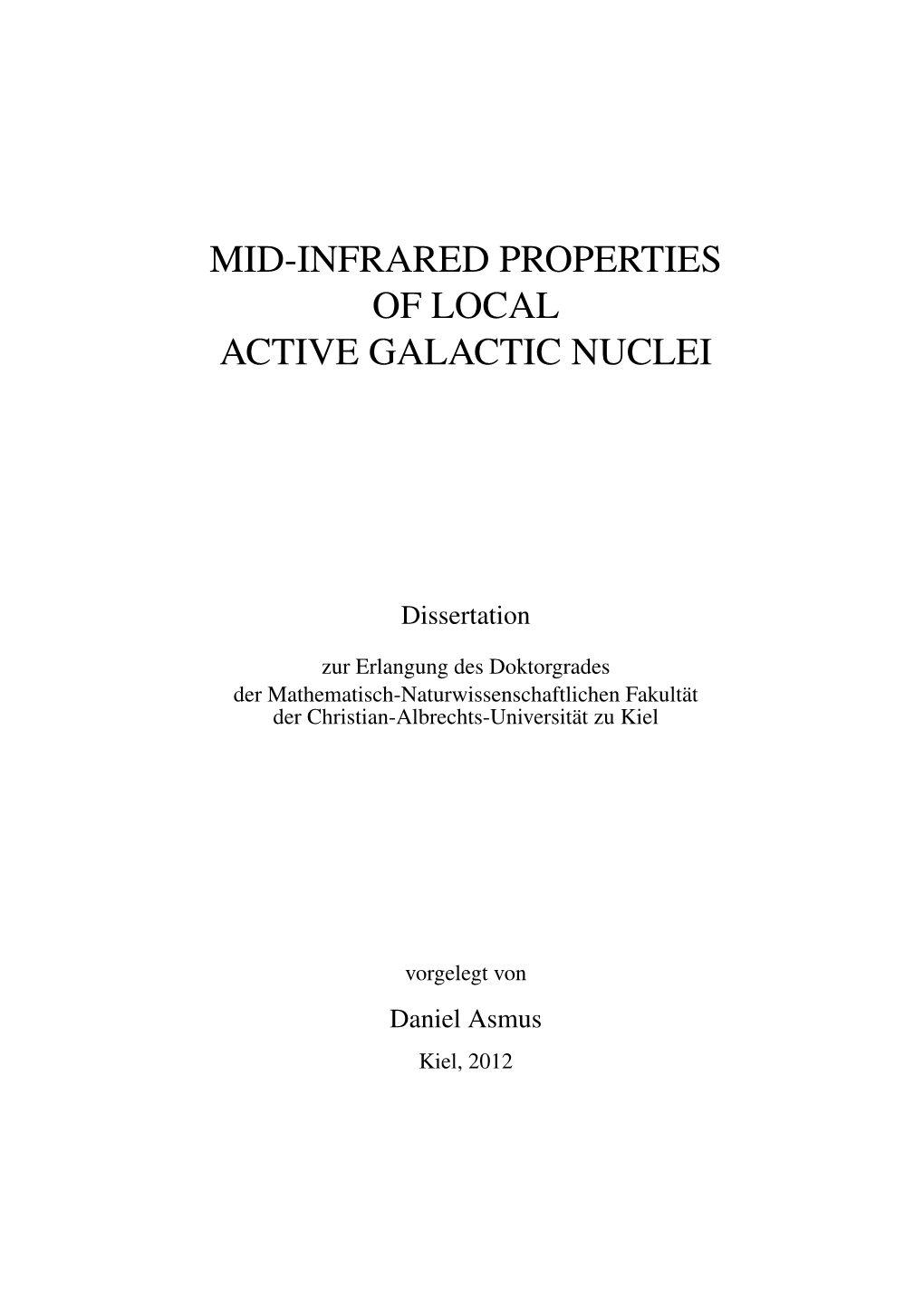
Load more
Recommended publications
-
Near-Infrared Luminosity Relations and Dust Colors L
A&A 578, A47 (2015) Astronomy DOI: 10.1051/0004-6361/201525817 & c ESO 2015 Astrophysics Obscuration in active galactic nuclei: near-infrared luminosity relations and dust colors L. Burtscher1, G. Orban de Xivry1, R. I. Davies1, A. Janssen1, D. Lutz1, D. Rosario1, A. Contursi1, R. Genzel1, J. Graciá-Carpio1, M.-Y. Lin1, A. Schnorr-Müller1, A. Sternberg2, E. Sturm1, and L. Tacconi1 1 Max-Planck-Institut für extraterrestrische Physik, Postfach 1312, Gießenbachstr., 85741 Garching, Germany e-mail: [email protected] 2 Raymond and Beverly Sackler School of Physics & Astronomy, Tel Aviv University, 69978 Ramat Aviv, Israel Received 5 February 2015 / Accepted 5 April 2015 ABSTRACT We combine two approaches to isolate the AGN luminosity at near-IR wavelengths and relate the near-IR pure AGN luminosity to other tracers of the AGN. Using integral-field spectroscopic data of an archival sample of 51 local AGNs, we estimate the fraction of non-stellar light by comparing the nuclear equivalent width of the stellar 2.3 µm CO absorption feature with the intrinsic value for each galaxy. We compare this fraction to that derived from a spectral decomposition of the integrated light in the central arcsecond and find them to be consistent with each other. Using our estimates of the near-IR AGN light, we find a strong correlation with presumably isotropic AGN tracers. We show that a significant offset exists between type 1 and type 2 sources in the sense that type 1 MIR X sources are 7 (10) times brighter in the near-IR at log LAGN = 42.5 (log LAGN = 42.5). -
Formation of an Ultra-Diffuse Galaxy in the Stellar Filaments of NGC 3314A
A&A 652, L11 (2021) Astronomy https://doi.org/10.1051/0004-6361/202141086 & c ESO 2021 Astrophysics LETTER TO THE EDITOR Formation of an ultra-diffuse galaxy in the stellar filaments of NGC 3314A: Caught in the act? Enrichetta Iodice1 , Antonio La Marca1, Michael Hilker2, Michele Cantiello3, Giuseppe D’Ago4, Marco Gullieuszik5, Marina Rejkuba2, Magda Arnaboldi2, Marilena Spavone1, Chiara Spiniello6, Duncan A. Forbes7, Laura Greggio5, Roberto Rampazzo5, Steffen Mieske8, Maurizio Paolillo9, and Pietro Schipani1 1 INAF-Astronomical Observatory of Capodimonte, Salita Moiariello 16, 80131 Naples, Italy e-mail: [email protected] 2 European Southern Observatory, Karl-Schwarzschild-Strasse 2, 85748 Garching bei Muenchen, Germany 3 INAF-Astronomical Observatory of Abruzzo, Via Maggini, 64100 Teramo, Italy 4 Instituto de Astrofísica, Facultad de Fisica, Pontificia Universidad Católica de Chile, Av. Vicuña Mackenna 4860, 7820436 Macul, Santiago, Chile 5 INAF-Osservatorio Astronomico di Padova, Vicolo dell’Osservatorio 5, 35122 Padova, Italy 6 Department of Physics, University of Oxford, Denys Wilkinson Building, Keble Road, Oxford OX1 3RH, UK 7 Centre for Astrophysics and Supercomputing, Swinburne University of Technology, Hawthorn, Victoria 3122, Australia 8 European Southern Observatory, Alonso de Cordova 3107, Vitacura, Santiago, Chile 9 University of Naples “Federico II”, C.U. Monte Sant’Angelo, Via Cinthia, 80126 Naples, Italy Received 14 April 2021 / Accepted 9 July 2021 ABSTRACT The VEGAS imaging survey of the Hydra I cluster has revealed an extended network of stellar filaments to the south-west of the spiral galaxy NGC 3314A. Within these filaments, at a projected distance of ∼40 kpc from the galaxy, we discover an ultra-diffuse galaxy −2 (UDG) with a central surface brightness of µ0;g ∼ 26 mag arcsec and effective radius Re ∼ 3:8 kpc. -
Probing the Active Galactic Nucleus Unified Model Torus Properties in Seyfert Galaxies
MNRAS 464, 2139–2173 (2017) doi:10.1093/mnras/stw2477 Advance Access publication 2016 September 30 Probing the active galactic nucleus unified model torus properties in Seyfert galaxies Anelise Audibert,1‹ Rogerio´ Riffel,1‹ Dinalva A. Sales,2 Miriani G. Pastoriza1 and Daniel Ruschel-Dutra1 1Departamento de Astronomia, Universidade Federal do Rio Grande do Sul, 9500 Bento Gonc¸alves, Porto Alegre 91501-970, Brazil 2Instituto de Matematica,´ Estat´ıstica e F´ısica, Universidade Federal do Rio Grande, Rio Grande 96203-900, Brazil Accepted 2016 September 28. Received 2016 September 27; in original form 2016 January 8 ABSTRACT We studied the physical parameters of a sample comprising of all Spitzer/Infrared Spectrograph public spectra of Seyfert galaxies in the mid-infrared (5.2–38 μm range) under the active 6 galactic nucleus (AGN) unified model. We compare the observed spectra with ∼10 CLUMPY model spectral energy distributions, which consider a torus composed of dusty clouds. We find a slight difference in the distribution of line-of-sight inclination angle, i, requiring larger angles for Seyfert 2 (Sy 2) and a broader distribution for Seyfert 1 (Sy 1). We found small differences in the torus angular width, σ , indicating that Sy 1 may host a slightly narrower torus than Sy 2. The torus thickness, together with the bolometric luminosities derived, suggests a very compact torus up to ∼6 pc from the central AGN. The number of clouds along the equatorial plane, N, as well the index of the radial profile, q, is nearly the same for both types. These results imply that the torus cloud distribution is nearly the same for type 1 and type 2 objects. -
Arxiv:0808.0461V1 [Astro-Ph] 4 Aug 2008
Draft version November 25, 2018 A Preprint typeset using LTEX style emulateapj v. 08/22/09 X-RAY SPECTRAL PROPERTIES OF THE BAT AGN SAMPLE Lisa M. Winter1, Richard F. Mushotzky2, Christopher S. Reynolds1, Jack Tueller2 Draft version November 25, 2018 ABSTRACT The 9-month SWIFT Burst Alert Telescope (BAT) catalog provides the first unbiased (NH < 1024 cm−2) look at local (<z>=0.03) AGN. In this paper, we present the collected X-ray properties (0.3 – 12 keV) for the 153 AGN detected. In addition, we examine the X-ray properties for a complete sample of non-beamed sources, above the Galactic plane (b 15◦). Of these, 45% are best fit by simple power law models while 55% require the more complex partial≥ covering model. One of our goals was to determine the fraction of “hidden” AGN, which we define as sources with scattering fractions 0.03 and ratios of soft to hard X-ray flux 0.04. We found that “hidden” AGN constitute a high percentage≤ of the sample (24%), proving that they≤ are a very significant portion of local AGN. Further, we find that the fraction of absorbed sources does increase at lower unabsorbed 2–10 keV luminosities, as well as accretion rates. This suggests that the unified model requires modification to include luminosity dependence, as suggested by models such as the ’receding torus’ model (Lawrence 1991). Some of the most interesting results for the BAT AGN sample involve the host galaxy properties. We found that 33% are hosted in peculiar/irregular galaxies and only 5/74 hosted in ellipticals. -
Lateinischer Name: Deutscher Name: Hya Hydra Wasserschlange
Lateinischer Name: Deutscher Name: Hya Hydra Wasserschlange Atlas Karte (2000.0) Kulmination um Cambridge 10, 16, Mitternacht: Star Atlas 17 12, 13, Sky Atlas Benachbarte Sternbilder: 20, 21 Ant Cnc Cen Crv Crt Leo Lib 9. Februar Lup Mon Pup Pyx Sex Vir Deklinationsbereic h: -35° ... 7° Fläche am Himmel: 1303° 2 Mythologie und Geschichte: Bei der nördlichen Wasserschlange überlagern sich zwei verschiedene Bilder aus der griechischen Mythologie. Das erste Bild zeugt von der eher harmlosen Wasserschlange aus der Geschichte des Raben : Der Rabe wurde von Apollon ausgesandt, um mit einem goldenen Becher frisches Quellwasser zu holen. Stattdessen tat sich dieser an Feigen gütlich und trug bei seiner Rückkehr die Wasserschlange in seinen Fängen, als angebliche Begründung für seine Verspätung. Um jedermann an diese Untat zu erinnern, wurden der Rabe samt Becher und Wasserschlange am Himmel zur Schau gestellt. Von einem ganz anderen Schlag war die Wasserschlange, mit der Herakles zu tun hatte: In einem Sumpf in der Nähe von Lerna, einem See und einer Stadt an der Küste von Argo, hauste ein unsagbar gefährliches und grässliches Untier. Diese Schlange soll mehrere Köpfe gehabt haben. Fünf sollen es gewesen sein, aber manche sprechen auch von sechs, neun, ja fünfzig oder hundert Köpfen, aber in jedem Falle war der Kopf in der Mitte unverwundbar. Fürchterlich war es, da diesen grässlichen Mäulern - ob die Schlange nun schlief oder wachte - ein fauliger Atem, ein Hauch entwich, dessen Gift tödlich war. Kaum schlug ein todesmutiger Mann dem Untier einen Kopf ab, wuchsen auf der Stelle zwei neue Häupter hervor, die noch furchterregender waren. Eurystheus, der König von Argos, beauftragte Herakles in seiner zweiten Aufgabe diese lernäische Wasserschlange zu töten. -
ATNF News Issue No
Galaxy Pair NGC 1512 / NGC 1510 ATNF News Issue No. 67, October 2009 ISSN 1323-6326 Questacon "astronaut" street performer and visitors at the Parkes Open Days 2009. Credit: Shaun Amy, CSIRO. Cover page image Cover Figure: Multi-wavelength color-composite image of the galaxy pair NGC 1512/1510 obtained using the Digitised Sky Survey R-band image (red), the Australia Telescope Compact Array HI distribution (green) and the Galaxy Evolution Explorer NUV -band image (blue). The Spitzer 24µm image was overlaid just in the center of the two galaxies. We note that in the outer disk the UV emission traces the regions of highest HI column density. See article (page 28) for more information. 2 ATNF News, Issue 67, October 2009 Contents From the Director ...................................................................................................................................................................................................4 CSIRO Medal Winners .........................................................................................................................................................................................5 CSIRO Astronomy and Space Science Unit Formed ........................................................................................................................6 ATNF Distinguished Visitors Program ........................................................................................................................................................6 ATNF Graduate Student Program ................................................................................................................................................................7 -
X-Ray Spectral Parameters for a Sample of 95 Active Galactic Nuclei
Accepted for publication in Astrophysics and Space Science X-Ray Spectral Parameters for a Sample of 95 Active Galactic Nuclei A.A. Vasylenko1, V.I. Zhdanov2, E.V. Fedorova2 1Main Astronomical Observatory NAS of Ukraine, Kyiv, Ukraine e-mail: [email protected] 2Taras Shevchenko National University of Kyiv, Ukraine We present a broadband X-ray analysis of a new homogeneous sample of 95 active galactic nuclei (AGN) from the 22-month Swift/BAT all-sky survey. For this sample we treated jointly the X-ray spectra observed by XMM-Newton and INTEGRAL missions for the total spectral range of 0.5-250 keV. Photon index Г, relative reflection R, equivalent width of Fe Kα line EWFeK, hydrogen column density NH, exponential cut-off energy Ec and intrinsic luminosity Lcorr are determined for all objects of the sample. We investigated correlations Г–R, EWFeK– Lcorr, Г–Ec, EWFeK–NH. Dependence "Г – R" for Seyfert ½ galaxies has been investigated separately. We found that the relative reflection parameter at low power-law indexes for Seyfert 2 galaxies is systematically higher than for Seyfert 1 ones. This can be related to an increasing contribution of the reflected radiation from the gas-dust torus. Our data show that there exists some anticorrelation between EWFeK and Lcorr, but it is not strong. We have not found statistically significant deviations from the AGN Unified Model. Key words: galaxies: Seyfert – X-rays: galaxies: active – galaxies 1. INTRODUCTION It is widely accepted today that the variety of observational appearances of AGNs can be divided in two big groups: those caused by physical conditions in particular AGN, and those due to geometrical effects of the AGN orientation with respect to the line-of-sight. -
Chandra LETGS Observation of the Variable NLS1 Galaxy Ark 564⋆
A&A 551, A95 (2013) Astronomy DOI: 10.1051/0004-6361/201220424 & c ESO 2013 Astrophysics Chandra LETGS observation of the variable NLS1 galaxy Ark 564 J. M. Ramírez Centro de Física, Instituto Venezolano de Investigaciones Científicas (IVIC), PO Box 025304, Caracas 1020A, Venezuela e-mail: [email protected] Received 21 September 2012 / Accepted 18 January 2013 ABSTRACT We present an analysis of the 100 ks X-ray spectrum of the narrow-line Seyfert 1 Galaxy Ark 564, taken with the Low Energy Transmission Grating Spectrometer (LETGS) on board Chandra.Usingχ2 statistics, several continuum models of the time-averaged spectrum of this object are compared, obtaining a semi-empirical solution for the description of the intrinsic emission continuum and a physical solution for the intrinsic absorption of the system. We find that the 0.1−10 keV spectrum can be well described by a power law plus two thermal components that account for the soft step. We are also able to detect and measure several narrow, unresolved absorption lines arising from highly ionized species of C, N, O, and Fe. The material seems to have a velocity consistent with the 20 −2 systemic velocity of the galaxy. This piece of evidence, in addition to the very low observed column density of NH ∼ 10 cm ,isin good agreement with the scenario of a transverse biconical outflow with a gas density of n 3 × 1012 cm−3 at distances beyond the broad-line region r 10 lt-days, but a dust torus origin cannot be ruled out. Key words. galaxies: Seyfert – line: identification – quasars: absorption lines 1. -
Arxiv:1004.5321V1 [Astro-Ph.CO] 29 Apr 2010 I-Nrrdpoete Fthe of Properties Mid-Infrared .A Evr .Mel´Endez M
Mid-Infrared Properties of the Swift Burst Alert Telescope Active Galactic Nuclei Sample of the Local Universe. I. Emission-Line Diagnostics K. A. Weaver, M. Mel´endez1,2, R. F. Mushotzky3, S. Kraemer4, K. Engle5, E. Malumuth6, J. Tueller, C. Markwardt3 NASA Goddard Space Flight Center, Greenbelt, MD, 20771 C.T. Berghea7 and R. P. Dudik U.S. Naval Observatory, Washington, DC 20392 L. M. Winter8 Center for Astrophysics and Space Astronomy, University of Colorado, Boulder, CO 80309-0440 and L. Armus Spitzer Science Center, California Institute of Technology, Pasadena, CA 91125 arXiv:1004.5321v1 [astro-ph.CO] 29 Apr 2010 –2– Received ; accepted To be submitted to the Astrophysical Journal 1NASA Postdoctoral Program Fellow, Goddard Space Flight Center, Greenbelt, MD, 20771 2present address: Department of Physics and Astronomy, Johns Hopkins University, Bal- timore, MD, 21218 3University of Maryland, College Park, MD 20742 4Institute for Astrophysics and Computational Sciences, Department of Physics, The Catholic University of America,Washington, DC 20064 5Adnet 6SESDA 7Computational Physics, Inc., Sprinfield, VA 22151 8Hubble Fellow –3– ABSTRACT We compare mid-infrared emission-line properties, from high-resolution Spitzer spectra of a hard X-ray (14 – 195 keV) selected sample of nearby (z < 0.05) AGN detected by the Burst Alert Telescope (BAT) aboard Swift. The lu- minosity distribution for the mid-infrared emission-lines, [O IV] 25.89 µm, [Ne II] 12.81 µm, [Ne III] 15.56 µm and [Ne V] 14.32/24.32 µm, and hard X-ray contin- uum show no differences between Seyfert 1 and Seyfert 2 populations, however six newly discovered BAT AGNs are under-luminous in [O IV], most likely the result of dust extinction in the host galaxy. -
Guide Du Ciel Profond
Guide du ciel profond Olivier PETIT 8 mai 2004 2 Introduction hjjdfhgf ghjfghfd fg hdfjgdf gfdhfdk dfkgfd fghfkg fdkg fhdkg fkg kfghfhk Table des mati`eres I Objets par constellation 21 1 Androm`ede (And) Andromeda 23 1.1 Messier 31 (La grande Galaxie d'Androm`ede) . 25 1.2 Messier 32 . 27 1.3 Messier 110 . 29 1.4 NGC 404 . 31 1.5 NGC 752 . 33 1.6 NGC 891 . 35 1.7 NGC 7640 . 37 1.8 NGC 7662 (La boule de neige bleue) . 39 2 La Machine pneumatique (Ant) Antlia 41 2.1 NGC 2997 . 43 3 le Verseau (Aqr) Aquarius 45 3.1 Messier 2 . 47 3.2 Messier 72 . 49 3.3 Messier 73 . 51 3.4 NGC 7009 (La n¶ebuleuse Saturne) . 53 3.5 NGC 7293 (La n¶ebuleuse de l'h¶elice) . 56 3.6 NGC 7492 . 58 3.7 NGC 7606 . 60 3.8 Cederblad 211 (N¶ebuleuse de R Aquarii) . 62 4 l'Aigle (Aql) Aquila 63 4.1 NGC 6709 . 65 4.2 NGC 6741 . 67 4.3 NGC 6751 (La n¶ebuleuse de l’œil flou) . 69 4.4 NGC 6760 . 71 4.5 NGC 6781 (Le nid de l'Aigle ) . 73 TABLE DES MATIERES` 5 4.6 NGC 6790 . 75 4.7 NGC 6804 . 77 4.8 Barnard 142-143 (La tani`ere noire) . 79 5 le B¶elier (Ari) Aries 81 5.1 NGC 772 . 83 6 le Cocher (Aur) Auriga 85 6.1 Messier 36 . 87 6.2 Messier 37 . 89 6.3 Messier 38 . -
A Basic Requirement for Studying the Heavens Is Determining Where In
Abasic requirement for studying the heavens is determining where in the sky things are. To specify sky positions, astronomers have developed several coordinate systems. Each uses a coordinate grid projected on to the celestial sphere, in analogy to the geographic coordinate system used on the surface of the Earth. The coordinate systems differ only in their choice of the fundamental plane, which divides the sky into two equal hemispheres along a great circle (the fundamental plane of the geographic system is the Earth's equator) . Each coordinate system is named for its choice of fundamental plane. The equatorial coordinate system is probably the most widely used celestial coordinate system. It is also the one most closely related to the geographic coordinate system, because they use the same fun damental plane and the same poles. The projection of the Earth's equator onto the celestial sphere is called the celestial equator. Similarly, projecting the geographic poles on to the celest ial sphere defines the north and south celestial poles. However, there is an important difference between the equatorial and geographic coordinate systems: the geographic system is fixed to the Earth; it rotates as the Earth does . The equatorial system is fixed to the stars, so it appears to rotate across the sky with the stars, but of course it's really the Earth rotating under the fixed sky. The latitudinal (latitude-like) angle of the equatorial system is called declination (Dec for short) . It measures the angle of an object above or below the celestial equator. The longitud inal angle is called the right ascension (RA for short). -
A New Sample of Buried Active Galactic Nuclei Selected from The
TO APPEAR IN The Astrophysical Journal. Preprint typeset using LATEX style emulateapj v. 08/22/09 A NEW SAMPLE OF BURIED ACTIVE GALACTIC NUCLEI SELECTED FROM THE SECOND XMM-NEWTON SERENDIPITOUS SOURCE CATALOGUE KAZUHISA NOGUCHI,YUICHI TERASHIMA, AND HISAMITSU AWAKI Department of Physics, Ehime University, Matsuyama, Ehime 790-8577, Japan To appear in The Astrophysical Journal. ABSTRACT We present the results of X-ray spectral analysis of 22 active galactic nuclei (AGNs) with a small scattering fraction selected from the Second XMM-Newton Serendipitous Source Catalogue using hardness ratios. They are candidates of buried AGNs, since a scattering fraction, which is a fraction of scattered emission by the circumnuclear photoionized gas with respect to direct emission, can be used to estimate the size of the opening part of an obscuring torus. Their X-ray spectra are modeled by a combination of a power law with a photon index of 1.5−2 absorbed by a column density of ∼ 1023−24 cm−2, an unabsorbed power law, narrow Gaussian lines, and some additional soft components. We find that scattering fractions of 20 among 22 objects are less than a typical value (∼ 3%) for Seyfert2s observed so far. In particular, those of eight objects are smaller than 0.5%, which are in the range for buried AGNs found in recent hard X-ray surveys. Moreover, [O III] λ5007 luminosities at given X-ray luminosities for some objects are smaller than those for Seyfert2s previouslyknown. This fact could be interpreted as a smaller size of optical narrow emission line regions produced in the opening direction of the obscuring torus.