NIH Public Access Author Manuscript J Gastroenterol
Total Page:16
File Type:pdf, Size:1020Kb
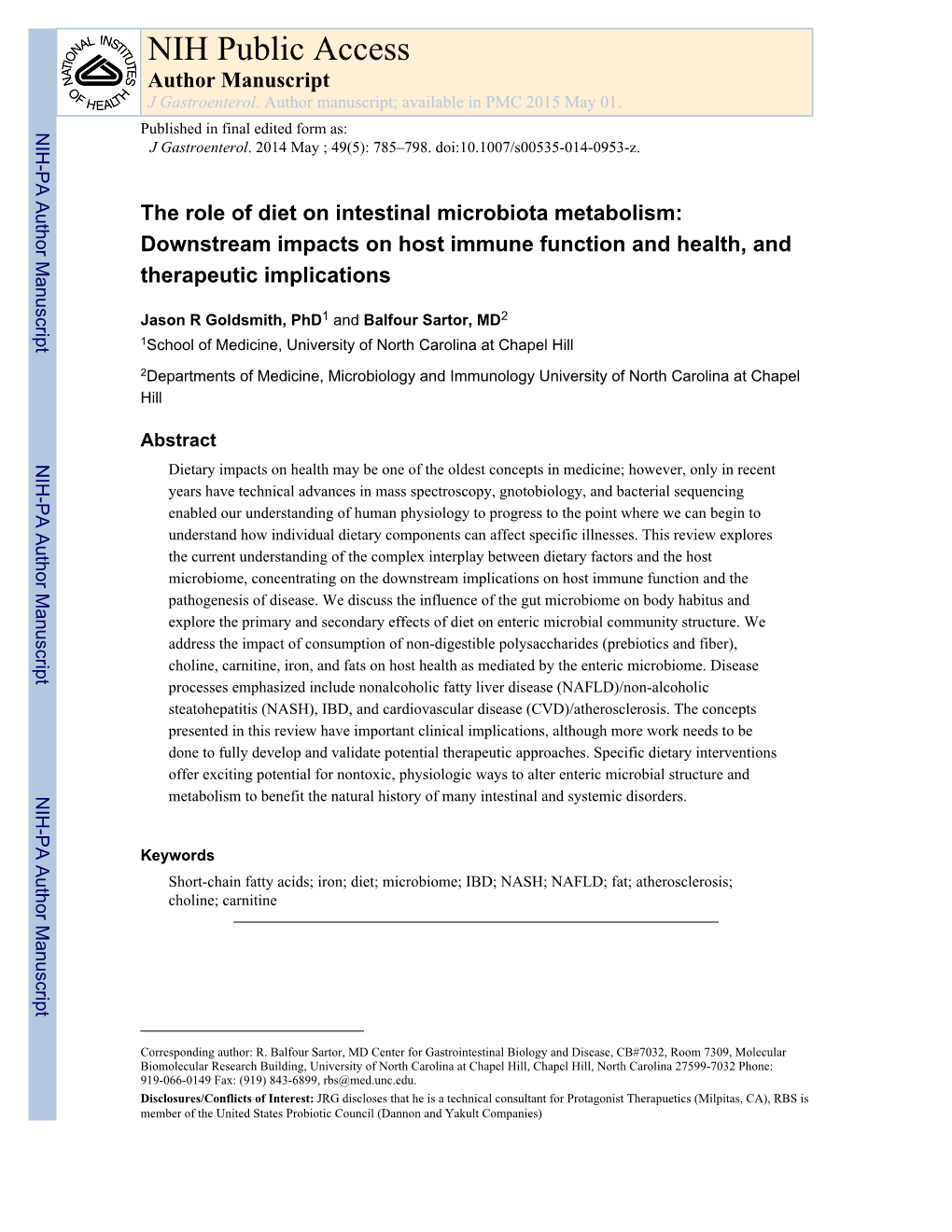
Load more
Recommended publications
-
Antioxidant Therapy in Inflammatory Bowel Diseases
antioxidants Review Antioxidant Therapy in Inflammatory Bowel Diseases Katarzyna Dzi ˛abowska-Grabias 1, Małgorzata Sztanke 2,* , Przemysław Zaj ˛ac 1, Michał Celejewski 1, Katarzyna Kurek 3, Stanisław Szkutnicki 3, Patryk Korga 4, Włodzimierz Bulikowski 5 and Krzysztof Sztanke 6 1 Department of Gastroenterology, 1st Military Research Hospital, and Polyclinic of Lublin, 20-049 Lublin, Poland; [email protected] (K.D.-G.); [email protected] (P.Z.); [email protected] (M.C.) 2 Department of Medical Chemistry, Medical University of Lublin, 20-093 Lublin, Poland 3 Department of Pneumonology, Oncology, and Allergology, Medical University of Lublin, 20-090 Lublin, Poland; [email protected] (K.K.); [email protected] (S.S.) 4 Department of Gastroenterology, 10ft Military Research Hospital, and Polyclinic of Bydgoszcz, 85-681 Bydgoszcz, Poland; [email protected] 5 Occupational Medicine Centre, 62-200 Gniezno, Poland; [email protected] 6 Laboratory of Bioorganic Synthesis and Analysis, Chair and Department of Medical Chemistry, Medical University of Lublin, 20-093 Lublin, Poland; [email protected] * Correspondence: [email protected]; Tel.: +48-814-486-195 Abstract: Inflammatory bowel diseases (IBD) are a group of chronic, incurable diseases of the diges- tive tract, the etiology of which remains unclear to this day. IBD result in significant repercussions on the quality of patients’ life. There is a continuous increase in the incidence and prevalence of IBD worldwide, and it is becoming a significant public health burden. Pharmaceuticals commonly used in IBD management, for example, mesalamine, sulfasalazine, corticosteroids, and others, expose patients to diverse, potentially detrimental side effects and frequently do not provide sufficient disease control. -
45 Diversion Colitis
45 Diversion colitis KONRAD H. SOERGEL Table 1. Possible etiologic mechanisms of diversion colitis Introduction [13] Diversion colitis is an inflammatory bowel disease Luminal short-chain fatty acid deficiency (IBD) that has attracted considerable clinical and Deficient short-chain fatty acid metabolism by colonocytes scientific interest during the past 15 years. Indeed, Pathogenic microorganisms - direct invasion or toxin elaboration the histologic features of this entity were recognized Stasis - bacterial overgrowth (similar to bypass enteritis) only 28 years ago [1] and in that time it has acquired Bile acid deficiency several names, including bypass colitis, exclusion colitis, and disuse colitis. Since its first clinical description in 1981 [2], a number of case reports and series have been published [2-30]. Certain observa tions have sparked the current curiosity: Pathophysiology/colonic SCFA 1. It is the only model of non-infectious 'experi mental' colitis in humans, with the possible ex metabolism ception of radiation colitis. It occurs in 50-100% While the clinical setting in which diversion colitis of cases following exclusion of the distal colo- develops is well estabhshed, little is known regarding rectum and is predictably reversible by surgical the etiologic mechanism. The sequence of events reanastomosis [2, 17, 18, 21]. following surgical bypass that culminates in inflam mation and clinical symptoms remains poorly under 2. It is caused by diversion of the fecal stream, most stood. Several theories have been proposed (Table 1). likely by deprivation of short-chain fatty acids Among these, only the virtual absence of SCFA from (SCFA) [13], the preferred metabolic substrate of the lumen of the bypassed segment is supported by the colonic epithelium [31-33]. -
Dietary and FODMAP Intake in Newly Diagnosed Inflammatory Bowel Disease Patients and Associations with Irritable Bowel Syndrome
Dietary and FODMAP intake in newly diagnosed inflammatory bowel disease patients and associations with irritable bowel syndrome Master thesis by Insaf Zerouga Supervisors: Monica H. Carlsen, Anne-Marie Aas and Christine Sommer Department of Nutrition, Faculty of Medicine UNIVERSITY OF OSLO May 2021 Abstract Introduction and aims: Inflammatory bowel disease (IBD), including Crohn’s disease (CD) and Ulcerative Colitis (UC) is often associated with high risk of malnutrition and multiple nutrient deficiencies. Dietary restrictions and modifications are common in IBD patients as an attempt to reduce gastrointestinal symptoms and improve health. These symptoms, also found in IBS patients, has been linked to dietary FODMAP intake. The evidence supporting this is growing, as clinical trials showed reduced IBS like symptoms with low FODMAP diet in IBD patients. However, Norwegian data on FODMAP content in foods is lacking. We aimed to compile FODMAP values in Norwegian foods, assess dietary intakes including FODMAPs in IBD patients and non-IBD controls, and examine the association between FODMAP in diet and IBS diagnosis in IBD patients. Method: The FODMAP compilation was based on previous analytical publications on FODMAP content in food. We included newly diagnosed, adult IBD patients (≥18 years) from the IBSEN III study recruited between January 1st 2017 and December 31st 2019. Dietary intake data were collected using a validated semi-quantitative, digital food frequency questionnaire (FFQ). Results: Dietary assessments were performed for a total of 779 participants with CD, UC and non-IBD controls. For both genders, intakes of saturated fat were higher, carbohydrates and vitamin D were lower, and in women iron was lower than recommended. -
Short Chain Fatty Acids As Potential Therapeutic Agents in Human Gastrointestinal and Inflammatory Disorders
Received: 20 December 2017 | First decision: 9 February 2018 | Accepted: 6 April 2018 DOI: 10.1111/apt.14689 Review article: short chain fatty acids as potential therapeutic agents in human gastrointestinal and inflammatory disorders P. A. Gill1,2 | M. C. van Zelm2 | J. G. Muir1 | P. R. Gibson1 1Department of Gastroenterology, Central Clinical School, Monash University and Summary Alfred Hospital, Melbourne, Vic., Australia Background: Butyrate, propionate and acetate are short chain fatty acids (SCFA), 2Department of Immunology and Pathology, important for maintaining a healthy colon and are considered as protective in col- Central Clinical School, Monash University and Alfred Hospital, Melbourne, Vic, orectal carcinogenesis. However, they may also regulate immune responses and the Australia composition of the intestinal microbiota. Consequently, their importance in a variety Correspondence of chronic inflammatory diseases is emerging. Prof. PR Gibson, Department of Aims: To review the physiology and metabolism of SCFA in humans, cellular and Gastroenterology, Central Clinical School, Monash University and Alfred Hospital, molecular mechanisms by which SCFA may act in health and disease, and Melbourne, Vic., Australia. approaches for therapeutic delivery of SCFA. Email: [email protected] Methods: A PubMed literature search was conducted for clinical and pre-clinical Funding information studies using search terms: ‘dietary fibre’, short-chain fatty acids’, ‘acetate’, ‘propi- PAG is supported by a scholarship from the Central Clinical School, Monash University. onate’, ‘butyrate’, ‘inflammation’, ‘immune’, ‘gastrointestinal’, ‘metabolism’. MCvZ is supported by NHMRC Senior Results: A wide range of pre-clinical evidence supports roles for SCFA as modula- Research Fellowship GNT1117687. JGM is supported by NHMRC Senior Research tors of not only colonic function, but also multiple inflammatory and metabolic pro- Fellowship GNT1136988. -
Diversion Colitis and Pouchitis: a Mini-Review
Submit a Manuscript: http://www.f6publishing.com World J Gastroenterol 2018 April 28; 24(16): 1734-1747 DOI: 10.3748/wjg.v24.i16.1734 ISSN 1007-9327 (print) ISSN 2219-2840 (online) MINIREVIEWS Diversion colitis and pouchitis: A mini-review Kentaro Tominaga, Kenya Kamimura, Kazuya Takahashi, Junji Yokoyama, Satoshi Yamagiwa, Shuji Terai Kentaro Tominaga, Kenya Kamimura, Kazuya Takahashi, Published online: April 28, 2018 Junji Yokoyama, Satoshi Yamagiwa, Shuji Terai, Division of Gastroenterology and Hepatology, Graduate School of Medical and Dental Sciences, Niigata University, Niigata 951-8510, Japan ORCID number: Kentaro Tominaga (0000-0001-6792-1005); Abstract Kenya Kamimura (0000-0001-7182-4400); Kazuya Takahashi Diversion colitis is characterized by inflammation of (0000-0002-3097-9841); Junji Yokoyama (0000-0002-1810 the mucosa in the defunctioned segment of the colon -7709); Satoshi Yamagiwa (0000-0003-4791-6107); Shuji Terai after colostomy or ileostomy. Similar to diversion colitis, (0000-0002-5439-635X). diversion pouchitis is an inflammatory disorder occurring Author contributions: Tominaga K and Kamimura K wrote the in the ileal pouch, resulting from the exclusion of the manuscript; Takahashi K, Yokoyama J, Yamagiwa S and Terai fecal stream and a subsequent lack of nutrients from S collected information; all authors read and approved the final luminal bacteria. Although the vast majority of patients version of the manuscript. with surgically-diverted gastrointestinal tracts remain asymptomatic, it has been reported that diversion Conflict-of-interest statement: The authors declare that they colitis and pouchitis might occur in almost all patients have no current financial arrangement or affiliation with any with diversion. Surgical closure of the stoma, with organization that may have a direct influence on their work. -
An Elemental Diet Is Effective in the Management of Diversion Colitis
astroenterology and Hepatology From Bed to Bench. CASE REPORT ©2021 RIGLD, Research Institute for Gastroenterology and Liver Diseases An elemental diet is effective in the management of diversion colitis Andrew Lane1, Nicholas Dalkie1, Lisa Henderson2, James Irwin1, Kamran Rostami1 1 Department of Gastroenterology, Palmerston North Hospital, New Zealand 2 Lisa Henderson,Dietetic/Nutrition, Palmerston North Hospital, New Zealand ABSTRACT The use of an elemental diet in the management of inflammatory gastroenterological diseases has long been accepted as standard management and has shown to both induce and maintain remission in Crohn’s disease, but the evidence is lacking for its use in diversion colitis. An elemental diet is one which provides all the required nutrition in a more easily absorbed and hypoallergenic form. In this case report, we present a patient who had a flare of diversion colitis treated with diet alone. She had a significant improvement in her symptoms with a decrease in bowel motions, rectal discharge and pain. This case suggests that there may be some role for the use of an elemental diet in the management of diversion colitis. We also examine some potential mechanisms which may lead to the benefit observed. Keywords: Elemental diet, Diversion colitis, Crohn’s disease, Ileostomy. (Please cite as: Lane A, Dalkie N, Henderson L, Irwin J, Rostami K. An elemental diet is effective in the management of diversion colitis. Gastroenterol Hepatol Bed Bench 2021;14(1):81-84). Introduction 1 The use of diet has long been widely accepted as an an ileostomy or colostomy formation (3,6). The most important part of standard management in effective management of these patients is surgical gastroenterological disorders, and an exclusive reversal of the stoma and regeneration of intestinal elemental diet has been shown to both induce and continuity. -
Diversion Colitis 25 Years Later: the Phenomenon of the Disease
Int J Colorectal Dis DOI 10.1007/s00384-017-2802-z ORIGINAL ARTICLE Diversion colitis 25 years later: the phenomenon of the disease Marek Szczepkowski 1,2 & Tomasz Banasiewicz3 & Adam Kobus1 Accepted: 23 March 2017 # The Author(s) 2017. This article is published with open access at Springerlink.com Abstract Conclusions The results of this study show a complex recur- Background Diversion colitis (DC) seems to be common in rence of histological inflammation several years after GI tract stoma patients, and the restoration of the continuity of the restoration but without clinical and endoscopic inflammation digestive tract is crucial for relief from the inflammatory pro- and with good clinical condition. DC can potentially have a cess. No prospective studies of the late effects of DC on the late influence on the rectal mucosa, even after stoma closure. lower gastrointestinal (GI) tract mucosa and the clinical con- dition of patients have been reported. Keywords Diversion colitis . Rectal stump . Inflammation . Methods Data from 23 patients who underwent stoma creation Mucosal proliferation were analysed during the reversal period (A) and at an average of 3 months (B1) and 5.6 years (B2) after restoration of GI tract continuity. Every monitoring visit included endoscopy, histol- ogy and assessment of the clinical condition of patients. Introduction Results Shortly after GI tract restoration (B1), a significant decrease in inflammation was observed. The Ki67 positivity Diversion colitis was described by Glotzer et al. in 1981 [1]. percentage increased, but this was not significant. At an aver- Twenty-five years ago, Harig et al. published the first report of age of 5.6 years after restoration (group B2), the clinical its treatment using short-chain fatty acids (SCFA) [2]. -
The Surgical Treatment of Acute and Severe Diversion Colitis Mimicking Ulcerative Colitis: a Case Report
Kakizawa et al. Surgical Case Reports (2018) 4:86 https://doi.org/10.1186/s40792-018-0490-8 CASEREPORT Open Access The surgical treatment of acute and severe diversion colitis mimicking ulcerative colitis: a case report Nao Kakizawa, Shingo Tsujinaka* , Yasuyuki Miyakura, Rina Kikugawa, Fumi Hasegawa, Hideki Ishikawa, Sawako Tamaki, Jun Takahashi and Toshiki Rikiyama Abstract Background: Diversion colitis (DC) is characterized by nonspecific inflammation in the remaining colon or rectum, and loss of the fecal stream plays a major role in the disease’s development. Although the majority of patients are asymptomatic, medical and/or surgical treatment is required for those who are symptomatic. There is a particular interest on how to manage patients with acute and severe clinical presentations, but the pathogenesis is not fully understood. We report the rare case of a man with acute and severe DC mimicking ulcerative colitis (UC) with extra-intestinal manifestations that was successfully managed with surgical treatment. Case presentation: A 68-year-old man with a history of laparoscopic intersphincteric resection of the rectum with diverting loop ileostomy for lower rectal cancer suffered from anastomotic stenosis requiring repeated endoscopic dilatation. His loop stoma was not reversed because these treatments were unsuccessful. He denied having a history of inflammatory bowel disease. Twelve years postoperatively, he developed a perineal abscess requiring drainage. Subsequently, he developed a high-grade fever, bloody discharge per anus, and skin ulcers in the right ankle and around the stoma. Because culture tests were negative for bacteria, it was deemed that his acute illness reflected an inflammatory response rather than an infectious disease. -
An Elemental Diet Is Effective in the Management of Diversion Colitis
Gastroenterology and Hepatology From Bed to Bench. CASE REPORT ©2021 RIGLD, Research Institute for Gastroenterology and Liver Diseases An elemental diet is effective in the management of diversion colitis Andrew Lane1, Nicholas Dalkie1, Lisa Henderson2, James Irwin1, Kamran Rostami1 1 Department of Gastroenterology, Palmerston North Hospital, New Zealand 2 Lisa Henderson,Dietetic/Nutrition, Palmerston North Hospital, New Zealand ABSTRACT The use of an elemental diet in the management of inflammatory gastroenterological diseases has long been accepted as standard management and has shown to both induce and maintain remission in Crohn’s disease, but the evidence is lacking for its use in diversion colitis. An elemental diet is one which provides all the required nutrition in a more easily absorbed and hypoallergenic form. In this case report, we present a patient who had a flare of diversion colitis treated with diet alone. She had a significant improvement in her symptoms with a decrease in bowel motions, rectal discharge and pain. This case suggests that there may be some role for the use of an elemental diet in the management of diversion colitis. We also examine some potential mechanisms which may lead to the benefit observed. Keywords: Elemental diet, Diversion colitis, Crohn’s disease, Ileostomy. (Please cite as: Lane A, Dalkie N, Henderson L, Irwin J, Rostami K. An elemental diet is effective in the management of diversion colitis. Gastroenterol Hepatol Bed Bench 2021;14(1):81-84). Introduction 1 The use of diet has long been widely accepted as an an ileostomy or colostomy formation (3,6). The most important part of standard management in effective management of these patients is surgical gastroenterological disorders, and an exclusive reversal of the stoma and regeneration of intestinal elemental diet has been shown to both induce and continuity. -
General Hospital Demographics
UK IBD Audit 2006 Executive Summary of the National Results for the Organisation & Process of IBD Care in the UK Prepared on behalf of The UK IBD Audit Steering Group by • Association of Coloproctology of Great Britain and Ireland • British Society of Gastroenterology • Clinical Effectiveness & Evaluation Unit, Royal College of Physicians of London • National Association of Colitis and Crohn’s Disease February 2007 REPORT PREPARED BY: Dr Keith Leiper Consultant Gastroenterologist, Royal Liverpool University Hospital & Clinical Director for the UK IBD Audit Mr Derek Lowe Medical Statistician, Clinical Effectiveness and Evaluation Unit, Royal College of Physicians Mr Richard Driscoll Director, National Association for Colitis and Crohn’s Disease (NACC) Miss Asha Senapati Consultant Surgeon, Queen Alexandra Hospital, Portsmouth Professor Jonathan Rhodes Professor of Medicine, University of Liverpool Mr Calvin Down IBD Audit Project Manager, Clinical Effectiveness and Evaluation Unit, Royal College of Physicians Miss Nancy Pursey IBD Audit Project Co-ordinator, Clinical Effectiveness and Evaluation Unit, Royal College of Physicians ACKNOWLEDGEMENTS The Royal College of Physicians of London and the UK IBD Audit Steering Group (Appendix 1) thank and acknowledge all who have participated in the piloting and development of the audit since the beginning of the Project. The web based data collection tool was developed by Netsolving Ltd. Thanks are due to the many people who have participated in the UK IBD Audit 2006. It is recognised that this has involved many individuals spending time over and above an already heavy workload with no financial recompense. Thanks are also due to • The Health Foundation who fund the UK IBD Audit • The Association of Coloproctology of Great Britain and Ireland • The British Society of Gastroenterology • The National Association for Colitis and Crohn’s Disease (NACC) • All those who contributed to organising the collection, retrieval and input of data including audit, IT and coding staff in addition to the members of the clinical teams.