MAP4K4 Expression in Cardiomyocytes: Multiple Isoforms, Multiple Phosphorylations and Interactions with Striatins
Total Page:16
File Type:pdf, Size:1020Kb
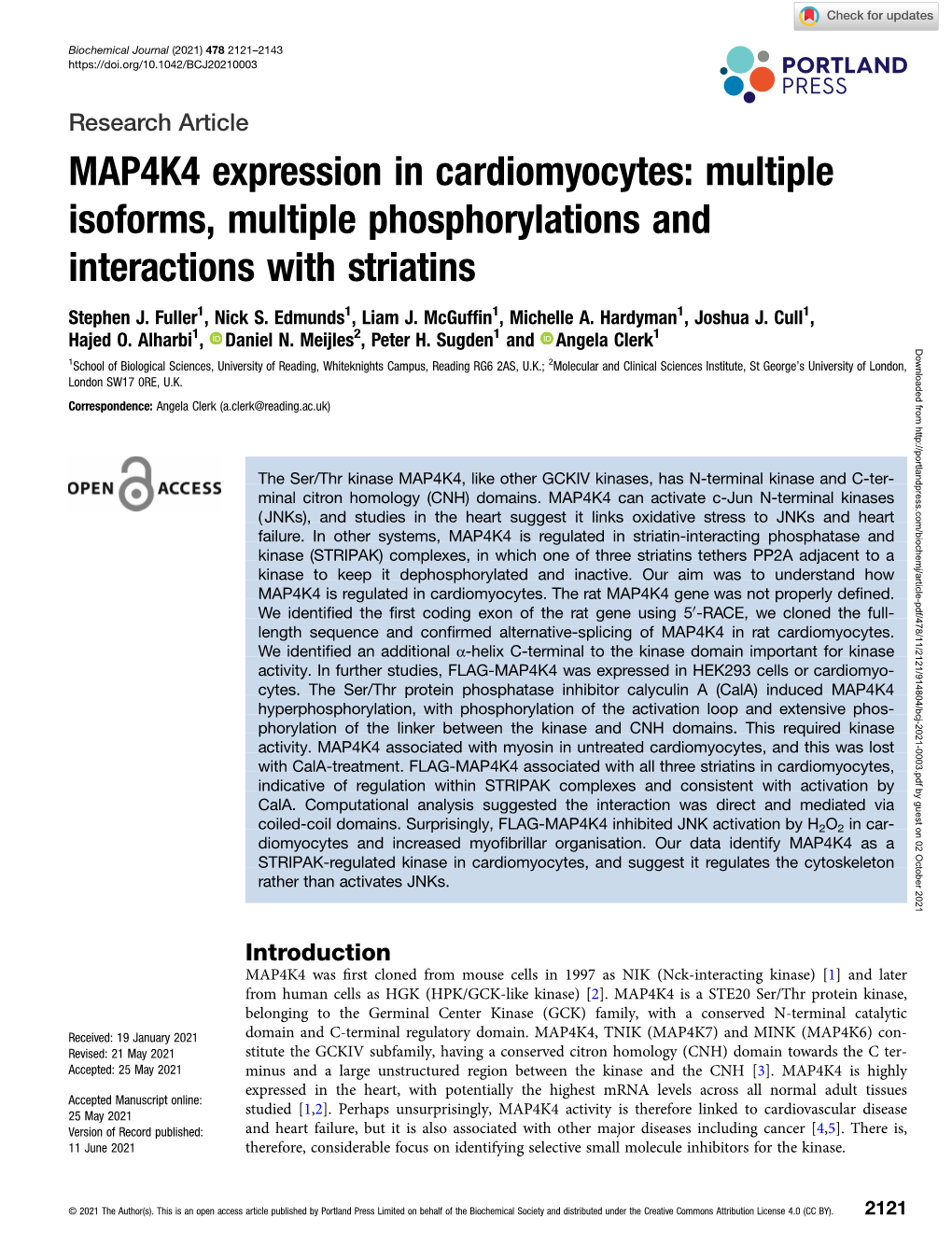
Load more
Recommended publications
-
Identification of the Transforming STRN-ALK Fusion As a Potential Therapeutic Target in the Aggressive Forms of Thyroid Cancer
Identification of the transforming STRN-ALK fusion as a potential therapeutic target in the aggressive forms of thyroid cancer Lindsey M. Kellya,1, Guillermo Barilab,1, Pengyuan Liuc,1, Viktoria N. Evdokimovaa, Sumita Trivedid, Federica Panebiancoa, Manoj Gandhia, Sally E. Cartye, Steven P. Hodakf, Jianhua Luoa, Sanja Dacica, Yan P. Yua, Marina N. Nikiforovaa, Robert L. Ferrisd, Daniel L. Altschulerb, and Yuri E. Nikiforova,2 aDepartment of Pathology and Laboratory Medicine, bDepartment of Pharmacology and Chemical Biology, dDepartment of Otolaryngology, eDepartment of Surgery, Division of Endocrine Surgery, and fDepartment of Medicine, Division of Endocrinology and Metabolism, University of Pittsburgh School of Medicine, Pittsburgh, PA 15213; and cDepartment of Physiology and Cancer Center, Medical College of Wisconsin, Milwaukee, WI 53226 Edited* by Albert de la Chapelle, Ohio State University Comprehensive Cancer Center, Columbus, OH, and approved January 10, 2014 (received for review November 24, 2013) Thyroid cancer is a common endocrine malignancy that encom- However, a significant proportion of thyroid cancers have no passes well-differentiated as well as dedifferentiated cancer types. known driver mutations. The discovery of novel genetic events The latter tumors have high mortality and lack effective therapies. has been accelerated more recently due to the availability of next- Using a paired-end RNA-sequencing approach, we report the dis- generation sequencing approaches that allow investigators to ob- covery of rearrangements involving the anaplastic lymphoma tain information on the entire genome, exome, or transcriptome kinase (ALK) gene in thyroid cancer. The most common of these of tumor cells (6). In this study, we used whole-transcriptome involves a fusion between ALK and the striatin (STRN) gene, which [RNA-sequencing (RNA-Seq)] analysis to identify novel gene fu- is the result of a complex rearrangement involving the short arm sions in thyroid cancer. -
Characterization of a Novel MAP4K4-SASH1 Kinase Cascade Regulating Breast Cancer Tumorigenesis and Metastasis
Characterization of a Novel MAP4K4-SASH1 Kinase Cascade Regulating Breast Cancer Tumorigenesis and Metastasis Yadong Li Guizhou Medical University Daoqiu Wu The Aliated Hospital of Guizhou Medical University Jing Hou Guizhou Provincial People's Hospital Jing Zhang The Aliated Hospital of Guizhou Medical University Xing Zeng Chongqing Medical University Lian Chen Guizhou Medical University Xin Wan Guizhou Medical University Zhixiong Wu Guizhou medical university Jinyun Wang Guizhou Medical University Ke Wang Yongchuan Hospital of Chongqing Dan Yang The Aliated Hospital of Guizhou Medical University Hongyu Chen Guizhou Medical University Zexi Xu Guizhou medical university Lei Jia Guizhou Medical University Qianfan Liu Guizhou medical university Zhongshu Kuang Page 1/30 Fudan university Geli Jiang Chongqing Cancer Hospital Hui Zhang Chongqing Zhongshan Hospital Jie Luo Chongqing Cancer Hospital Wei Li Chongqing Cancer Hospital Xue Zou The Aliated Hospital of Guizhou Medical University Xiaohua Zeng Chongqing Cancer Hospital Ding'an Zhou ( [email protected] ) Guizhou Medical University https://orcid.org/0000-0002-6614-9321 Research Keywords: SASH1, MAP4K4, Tumorigenesis, Metastasis, Hormone-dependent breast cancers Posted Date: November 5th, 2020 DOI: https://doi.org/10.21203/rs.3.rs-101160/v1 License: This work is licensed under a Creative Commons Attribution 4.0 International License. Read Full License Page 2/30 Abstract Background: The SAM and SH3 domain containing protein 1(SASH1) was previously described as a candidate tumor-suppressor gene in breast cancer and colon cancer to mediate tumor metastasis and tumor growth. Howeverthe underlying mechanisms by which SASH1 implements breast cancer tumorigenesis and the question why SASH1 is downregulated in most solid cancers remain unexplored. -
MAP4K Family Kinases Act in Parallel to MST1/2 to Activate LATS1/2 in the Hippo Pathway
ARTICLE Received 19 Jun 2015 | Accepted 13 Aug 2015 | Published 5 Oct 2015 DOI: 10.1038/ncomms9357 OPEN MAP4K family kinases act in parallel to MST1/2 to activate LATS1/2 in the Hippo pathway Zhipeng Meng1, Toshiro Moroishi1, Violaine Mottier-Pavie2, Steven W. Plouffe1, Carsten G. Hansen1, Audrey W. Hong1, Hyun Woo Park1, Jung-Soon Mo1, Wenqi Lu1, Shicong Lu1, Fabian Flores1, Fa-Xing Yu3, Georg Halder2 & Kun-Liang Guan1 The Hippo pathway plays a central role in tissue homoeostasis, and its dysregulation contributes to tumorigenesis. Core components of the Hippo pathway include a kinase cascade of MST1/2 and LATS1/2 and the transcription co-activators YAP/TAZ. In response to stimulation, LATS1/2 phosphorylate and inhibit YAP/TAZ, the main effectors of the Hippo pathway. Accumulating evidence suggests that MST1/2 are not required for the regulation of YAP/TAZ. Here we show that deletion of LATS1/2 but not MST1/2 abolishes YAP/TAZ phosphorylation. We have identified MAP4K family members—Drosophila Happyhour homologues MAP4K1/2/3 and Misshapen homologues MAP4K4/6/7—as direct LATS1/2-activating kinases. Combined deletion of MAP4Ks and MST1/2, but neither alone, suppresses phosphorylation of LATS1/2 and YAP/TAZ in response to a wide range of signals. Our results demonstrate that MAP4Ks act in parallel to and are partially redundant with MST1/2 in the regulation of LATS1/2 and YAP/TAZ, and establish MAP4Ks as components of the expanded Hippo pathway. 1 Department of Pharmacology and Moores Cancer Center, University of California San Diego, La Jolla, California 92093, USA. -
MAP4K4 Expression in Cardiomyocytes: Multiple Isoforms, Multiple Phosphorylations and Interactions with Striatins
MAP4K4 expression in cardiomyocytes: multiple isoforms, multiple phosphorylations and interactions with striatins Article Published Version Creative Commons: Attribution 4.0 (CC-BY) Open access Fuller, S. J., Edmunds, N. S., McGuffin, L. J. ORCID: https://orcid.org/0000-0003-4501-4767, Hardyman, M. A., Cull, J. J., Alharbi, H. O., Meijles, D. N., Sugden, P. H. and Clerk, A. ORCID: https://orcid.org/0000-0002-5658-0708 (2021) MAP4K4 expression in cardiomyocytes: multiple isoforms, multiple phosphorylations and interactions with striatins. Biochemical Journal, 478 (11). pp. 2121-2143. ISSN 0264- 6021 doi: https://doi.org/10.1042/BCJ20210003 Available at http://centaur.reading.ac.uk/98442/ It is advisable to refer to the publisher’s version if you intend to cite from the work. See Guidance on citing . Published version at: http://dx.doi.org/10.1042/BCJ20210003 To link to this article DOI: http://dx.doi.org/10.1042/BCJ20210003 Publisher: Portland Press All outputs in CentAUR are protected by Intellectual Property Rights law, including copyright law. Copyright and IPR is retained by the creators or other copyright holders. Terms and conditions for use of this material are defined in the End User Agreement . www.reading.ac.uk/centaur CentAUR Central Archive at the University of Reading Reading’s research outputs online Biochemical Journal (2021) 478 2121–2143 https://doi.org/10.1042/BCJ20210003 Research Article MAP4K4 expression in cardiomyocytes: multiple isoforms, multiple phosphorylations and interactions with striatins Stephen J. Fuller1, Nick S. Edmunds1, Liam J. McGuffin1, Michelle A. Hardyman1, Joshua J. Cull1, Hajed O. Alharbi1, Daniel N. Meijles2, Peter H. -
Supplementary Table S1. Upregulated Genes Differentially
Supplementary Table S1. Upregulated genes differentially expressed in athletes (p < 0.05 and 1.3-fold change) Gene Symbol p Value Fold Change 221051_s_at NMRK2 0.01 2.38 236518_at CCDC183 0.00 2.05 218804_at ANO1 0.00 2.05 234675_x_at 0.01 2.02 207076_s_at ASS1 0.00 1.85 209135_at ASPH 0.02 1.81 228434_at BTNL9 0.03 1.81 229985_at BTNL9 0.01 1.79 215795_at MYH7B 0.01 1.78 217979_at TSPAN13 0.01 1.77 230992_at BTNL9 0.01 1.75 226884_at LRRN1 0.03 1.74 220039_s_at CDKAL1 0.01 1.73 236520_at 0.02 1.72 219895_at TMEM255A 0.04 1.72 201030_x_at LDHB 0.00 1.69 233824_at 0.00 1.69 232257_s_at 0.05 1.67 236359_at SCN4B 0.04 1.64 242868_at 0.00 1.63 1557286_at 0.01 1.63 202780_at OXCT1 0.01 1.63 1556542_a_at 0.04 1.63 209992_at PFKFB2 0.04 1.63 205247_at NOTCH4 0.01 1.62 1554182_at TRIM73///TRIM74 0.00 1.61 232892_at MIR1-1HG 0.02 1.61 204726_at CDH13 0.01 1.6 1561167_at 0.01 1.6 1565821_at 0.01 1.6 210169_at SEC14L5 0.01 1.6 236963_at 0.02 1.6 1552880_at SEC16B 0.02 1.6 235228_at CCDC85A 0.02 1.6 1568623_a_at SLC35E4 0.00 1.59 204844_at ENPEP 0.00 1.59 1552256_a_at SCARB1 0.02 1.59 1557283_a_at ZNF519 0.02 1.59 1557293_at LINC00969 0.03 1.59 231644_at 0.01 1.58 228115_at GAREM1 0.01 1.58 223687_s_at LY6K 0.02 1.58 231779_at IRAK2 0.03 1.58 243332_at LOC105379610 0.04 1.58 232118_at 0.01 1.57 203423_at RBP1 0.02 1.57 AMY1A///AMY1B///AMY1C///AMY2A///AMY2B// 208498_s_at 0.03 1.57 /AMYP1 237154_at LOC101930114 0.00 1.56 1559691_at 0.01 1.56 243481_at RHOJ 0.03 1.56 238834_at MYLK3 0.01 1.55 213438_at NFASC 0.02 1.55 242290_at TACC1 0.04 1.55 ANKRD20A1///ANKRD20A12P///ANKRD20A2/// -
Mediator of DNA Damage Checkpoint 1 (MDC1) Is a Novel Estrogen Receptor Co-Regulator in Invasive 6 Lobular Carcinoma of the Breast 7 8 Evelyn K
bioRxiv preprint doi: https://doi.org/10.1101/2020.12.16.423142; this version posted December 16, 2020. The copyright holder for this preprint (which was not certified by peer review) is the author/funder, who has granted bioRxiv a license to display the preprint in perpetuity. It is made available under aCC-BY-NC 4.0 International license. 1 Running Title: MDC1 co-regulates ER in ILC 2 3 Research article 4 5 Mediator of DNA damage checkpoint 1 (MDC1) is a novel estrogen receptor co-regulator in invasive 6 lobular carcinoma of the breast 7 8 Evelyn K. Bordeaux1+, Joseph L. Sottnik1+, Sanjana Mehrotra1, Sarah E. Ferrara2, Andrew E. Goodspeed2,3, James 9 C. Costello2,3, Matthew J. Sikora1 10 11 +EKB and JLS contributed equally to this project. 12 13 Affiliations 14 1Dept. of Pathology, University of Colorado Anschutz Medical Campus 15 2Biostatistics and Bioinformatics Shared Resource, University of Colorado Comprehensive Cancer Center 16 3Dept. of Pharmacology, University of Colorado Anschutz Medical Campus 17 18 Corresponding author 19 Matthew J. Sikora, PhD.; Mail Stop 8104, Research Complex 1 South, Room 5117, 12801 E. 17th Ave.; Aurora, 20 CO 80045. Tel: (303)724-4301; Fax: (303)724-3712; email: [email protected]. Twitter: 21 @mjsikora 22 23 Authors' contributions 24 MJS conceived of the project. MJS, EKB, and JLS designed and performed experiments. JLS developed models 25 for the project. EKB, JLS, SM, and AEG contributed to data analysis and interpretation. SEF, AEG, and JCC 26 developed and performed informatics analyses. MJS wrote the draft manuscript; all authors read and revised the 27 manuscript and have read and approved of this version of the manuscript. -
High-Throughput Discovery of Novel Developmental Phenotypes
High-throughput discovery of novel developmental phenotypes The Harvard community has made this article openly available. Please share how this access benefits you. Your story matters Citation Dickinson, M. E., A. M. Flenniken, X. Ji, L. Teboul, M. D. Wong, J. K. White, T. F. Meehan, et al. 2016. “High-throughput discovery of novel developmental phenotypes.” Nature 537 (7621): 508-514. doi:10.1038/nature19356. http://dx.doi.org/10.1038/nature19356. Published Version doi:10.1038/nature19356 Citable link http://nrs.harvard.edu/urn-3:HUL.InstRepos:32071918 Terms of Use This article was downloaded from Harvard University’s DASH repository, and is made available under the terms and conditions applicable to Other Posted Material, as set forth at http:// nrs.harvard.edu/urn-3:HUL.InstRepos:dash.current.terms-of- use#LAA HHS Public Access Author manuscript Author ManuscriptAuthor Manuscript Author Nature. Manuscript Author Author manuscript; Manuscript Author available in PMC 2017 March 14. Published in final edited form as: Nature. 2016 September 22; 537(7621): 508–514. doi:10.1038/nature19356. High-throughput discovery of novel developmental phenotypes A full list of authors and affiliations appears at the end of the article. Abstract Approximately one third of all mammalian genes are essential for life. Phenotypes resulting from mouse knockouts of these genes have provided tremendous insight into gene function and congenital disorders. As part of the International Mouse Phenotyping Consortium effort to generate and phenotypically characterize 5000 knockout mouse lines, we have identified 410 Users may view, print, copy, and download text and data-mine the content in such documents, for the purposes of academic research, subject always to the full Conditions of use:http://www.nature.com/authors/editorial_policies/license.html#terms #Corresponding author: [email protected]. -
The MAP4K4-STRIPAK Complex Promotes Growth and Tissue Invasion In
bioRxiv preprint doi: https://doi.org/10.1101/2021.05.07.442906; this version posted May 8, 2021. The copyright holder for this preprint (which was not certified by peer review) is the author/funder. All rights reserved. No reuse allowed without permission. 1 The MAP4K4-STRIPAK complex promotes growth and tissue invasion in 2 medulloblastoma 3 Jessica Migliavacca1, Buket Züllig1, Charles Capdeville1, Michael Grotzer2 and Martin Baumgartner1,* 4 1 Division of Oncology, Children’s Research Center, University Children’s Hospital Zürich, Zürich, 5 Switzerland 6 2 Division of Oncology, University Children’s Hospital Zürich, Zürich, Switzerland 7 8 *e-mail: [email protected] 9 10 Abstract 11 Proliferation and motility are mutually exclusive biological processes associated with cancer that depend 12 on precise control of upstream signaling pathways with overlapping functionalities. We find that STRN3 13 and STRN4 scaffold subunits of the STRIPAK complex interact with MAP4K4 for pathway regulation in 14 medulloblastoma. Disruption of the MAP4K4-STRIPAK complex impairs growth factor-induced 15 migration and tissue invasion and stalls YAP/TAZ target gene expression and oncogenic growth. The 16 migration promoting functions of the MAP4K4-STRIPAK complex involve the activation of novel PKCs 17 and the phosphorylation of the membrane targeting S157 residue of VASP through MAP4K4. The anti- 18 proliferative effect of complex disruption is associated with reduced YAP/TAZ target gene expression 19 and results in repressed tumor growth in the brain tissue. This dichotomous functionality of the STRIPAK 20 complex in migration and proliferation control acts through MAP4K4 regulation in tumor cells and 21 provides relevant mechanistic insights into novel tumorigenic functions of the STRIPAK complex in 22 medulloblastoma. -
Application of a MYC Degradation
SCIENCE SIGNALING | RESEARCH ARTICLE CANCER Copyright © 2019 The Authors, some rights reserved; Application of a MYC degradation screen identifies exclusive licensee American Association sensitivity to CDK9 inhibitors in KRAS-mutant for the Advancement of Science. No claim pancreatic cancer to original U.S. Devon R. Blake1, Angelina V. Vaseva2, Richard G. Hodge2, McKenzie P. Kline3, Thomas S. K. Gilbert1,4, Government Works Vikas Tyagi5, Daowei Huang5, Gabrielle C. Whiten5, Jacob E. Larson5, Xiaodong Wang2,5, Kenneth H. Pearce5, Laura E. Herring1,4, Lee M. Graves1,2,4, Stephen V. Frye2,5, Michael J. Emanuele1,2, Adrienne D. Cox1,2,6, Channing J. Der1,2* Stabilization of the MYC oncoprotein by KRAS signaling critically promotes the growth of pancreatic ductal adeno- carcinoma (PDAC). Thus, understanding how MYC protein stability is regulated may lead to effective therapies. Here, we used a previously developed, flow cytometry–based assay that screened a library of >800 protein kinase inhibitors and identified compounds that promoted either the stability or degradation of MYC in a KRAS-mutant PDAC cell line. We validated compounds that stabilized or destabilized MYC and then focused on one compound, Downloaded from UNC10112785, that induced the substantial loss of MYC protein in both two-dimensional (2D) and 3D cell cultures. We determined that this compound is a potent CDK9 inhibitor with a previously uncharacterized scaffold, caused MYC loss through both transcriptional and posttranslational mechanisms, and suppresses PDAC anchorage- dependent and anchorage-independent growth. We discovered that CDK9 enhanced MYC protein stability 62 through a previously unknown, KRAS-independent mechanism involving direct phosphorylation of MYC at Ser . -
Transcriptome Analysis of Human Diabetic Kidney Disease
ORIGINAL ARTICLE Transcriptome Analysis of Human Diabetic Kidney Disease Karolina I. Woroniecka,1 Ae Seo Deok Park,1 Davoud Mohtat,2 David B. Thomas,3 James M. Pullman,4 and Katalin Susztak1,5 OBJECTIVE—Diabetic kidney disease (DKD) is the single cases, mild and then moderate mesangial expansion can be leading cause of kidney failure in the U.S., for which a cure has observed. In general, diabetic kidney disease (DKD) is not yet been found. The aim of our study was to provide an considered a nonimmune-mediated degenerative disease unbiased catalog of gene-expression changes in human diabetic of the glomerulus; however, it has long been noted that kidney biopsy samples. complement and immunoglobulins sometimes can be de- — tected in diseased glomeruli, although their role and sig- RESEARCH DESIGN AND METHODS Affymetrix expression fi arrays were used to identify differentially regulated transcripts in ni cance is not clear (4). 44 microdissected human kidney samples. The DKD samples were The understanding of DKD has been challenged by multi- significant for their racial diversity and decreased glomerular ple issues. First, the diagnosis of DKD usually is made using filtration rate (~20–30 mL/min). Stringent statistical analysis, using clinical criteria, and kidney biopsy often is not performed. the Benjamini-Hochberg corrected two-tailed t test, was used to According to current clinical practice, the development of identify differentially expressed transcripts in control and diseased albuminuria in patients with diabetes is sufficient to make the glomeruli and tubuli. Two different Web-based algorithms were fi diagnosis of DKD (5). We do not understand the correlation used to de ne differentially regulated pathways. -
Non-Target Genes Regulate Mirnas-Mediated Migration Steering of Colorectal Carcinoma
Pathology & Oncology Research (2019) 25:559–566 https://doi.org/10.1007/s12253-018-0502-9 ORIGINAL ARTICLE Non-target Genes Regulate miRNAs-Mediated Migration Steering of Colorectal Carcinoma Sohair M. Salem1 & Ahmed R. Hamed2,3 & Alaaeldin G. Fayez1 & Ghada Nour Eldeen1,4 Received: 20 January 2018 /Accepted: 15 October 2018 /Published online: 25 October 2018 # Arányi Lajos Foundation 2018 Abstract MicroRNAs (miRNAs) trigger a two-layer regulatory network directly or through transcription factors and their co-regulators. Unlike miR-375, the role of miR-145 and miR-224 in inhibiting or driving cancer cell migration is controversial. This study is a step towards addressing the potential of miR-375, miR-145 and miR-224 expression modulation to inhibit colorectal carcinoma (CRC) cells migration in vitro through regulation of non-target genes VEGFA, TGFβ1, IGF1, CD105 and CD44. Transwell migration assay results revealed a significant subdue of migration ability of cells transfected with miR-375 and miR-145 mimics and miR-224 inhibitor. Real time PCR data showed that expression of VEGFA, TGFβ1, IGF1, CD105 and CD44 was downreg- ulated as a consequence of exogenous re-expression of miR-375 and inhibition of miR-224. On the other hand, ectopic expression of miR-145 did not affect VEGFA, TGFβ1 and CD44 expression, while it elevated CD105 and suppressed IGF1 expression. MAP4K4, a predicted target of miR-145, was validated as a target that could play a role in miR-145-mediated regulation of migration. At mRNA level, no change was observed in expression of MAP4K4 in cells with restored expression of miR-145, while western blotting analysis revealed a 25% reduction of protein level. -
Loss of PPP2R2A Inhibits Homologous Recombination DNA Repair and Predicts
Author Manuscript Published OnlineFirst on October 18, 2012; DOI: 10.1158/0008-5472.CAN-12-1667 Author manuscripts have been peer reviewed and accepted for publication but have not yet been edited. Loss of PPP2R2A inhibits homologous recombination DNA repair and predicts tumor sensitivity to PARP inhibition Peter Kalev1, Michal Simicek1, Iria Vazquez1, Sebastian Munck1, Liping Chen2, Thomas Soin1, Natasha Danda1, Wen Chen2 and Anna Sablina1,* 1VIB Center for the Biology of Disease; Center for Human Genetics, KULeuven, Leuven 3000 Belgium 2Department of Toxicology, Faculty of Preventive Medicine, Guangdong Provincial Key Laboratory of Food, Nutrition and Health, School of Public Health, Sun Yat-sen University, Guangzhou 510080, China *Corresponding author information: [email protected] Contact information: Anna A. Sablina, Ph.D. CME Department, KULeuven O&N I Herestraat 49, bus 602 Leuven, Belgium 3000 Tel: +3216330790 Fax: +3216330145 Running title: The role of PPP2R2A in DNA repair Keywords: PP2A, ATM, DNA repair, cancer, PARP inhibition Conflict of interests: The authors claim no conflict of interest. - 1 - Downloaded from cancerres.aacrjournals.org on September 27, 2021. © 2012 American Association for Cancer Research. Author Manuscript Published OnlineFirst on October 18, 2012; DOI: 10.1158/0008-5472.CAN-12-1667 Author manuscripts have been peer reviewed and accepted for publication but have not yet been edited. Abstract Reversible phosphorylation plays a critical role in DNA repair. Here we report the results of a loss-of-function screen that identifies the PP2A heterotrimeric serine/threonine phosphatases PPP2R2A, PPP2R2D, PPP2R5A and PPP2R3C in double-strand break (DSB) repair. In particular, we found that PPP2R2A-containing complexes directly dephosphorylated ATM at S367, S1893, and S1981 to regulate its retention at DSB sites.