Intrinsic Bitterness of Flavonoids and Isoflavonoids and Masking of Their Taste Activity
Total Page:16
File Type:pdf, Size:1020Kb
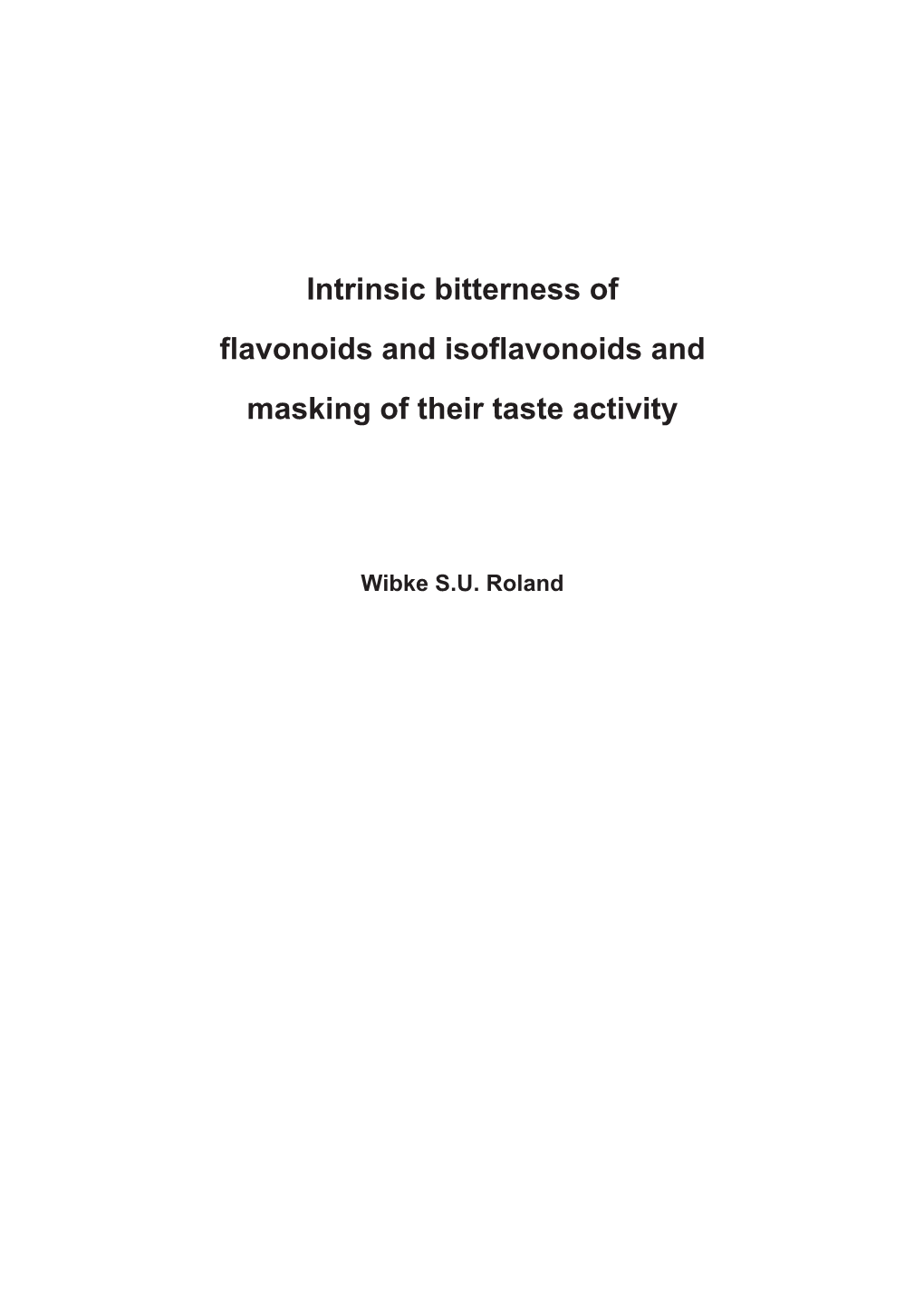
Load more
Recommended publications
-
Boards' Fodder
boards’ fodder Cosmeceuticals Contributed by Elisabeth Hurliman, MD, PhD; Jennifer Hayes, MD; Hilary Reich MD; and Sarah Schram, MD. INGREDIENT FUNCTION MECHANISM ASSOCIATIONS/SIDE EFFECTS Vitamin A/ Antioxidant (reduces free Affects gene transcription Comedolysis epidermal thickening, dermal Derivatives (retinal, radicals, lowers concentration differentiation and growth of regeneration, pigment lightening retinol, retinoic of matrix metalloproteinases cells in the skin acid, provitamin reduces collagen degradation) Side effects: Irritation, erythema, desquamation A, asthaxanthin, Normalizes follicular Elisabeth Hurliman, lutein) epithelial differentiation and keratinization MD, PhD, is a PGY-4 dermatology resident Vitamin C (L Secondary endogenous Ascorbic acid: necessary L-ascorbic acid + alpha-tocopherol (vitamin E)= ascorbic acid, antioxidant in skin cofactor for prolylhydroxylase UVA and UVB protection at University of tetrahexyldecyl and lysyl hydroxylase Minnesota department ascorbate) Lightens pigment Zinc, resveratrol, L-ergothioneine and tyrosine add of dermatology. (affects melanogenesis) L-ascorbic acid: scavenges to vitamin C bioavailability free oxygen radicals, Protects Vitamin E from oxidation stimulates collagen synthesis Improves skin texture and hydration May interrupt melanogenesis by interacting with copper ions Vitamin E/ Primary endogenous antioxidant Prevents lipid peroxidation; Alpha tocopherol is the most physiologically Tocopherols, in skin scavenges free oxygen active isomer Jennifer Hayes, MD, Tocotrienols -
LC-MS Profile, Gastrointestinal and Gut Microbiota
antioxidants Article LC-MS Profile, Gastrointestinal and Gut Microbiota Stability and Antioxidant Activity of Rhodiola rosea Herb Metabolites: A Comparative Study with Subterranean Organs Daniil N. Olennikov 1,* , Nadezhda K. Chirikova 2, Aina G. Vasilieva 2 and Innokentii A. Fedorov 3 1 Laboratory of Medical and Biological Research, Institute of General and Experimental Biology, Siberian Division, Russian Academy of Science, 6 Sakh’yanovoy Street, Ulan-Ude 670047, Russia 2 Department of Biology, Institute of Natural Sciences, North-Eastern Federal University, 58 Belinsky Street, Yakutsk 677027, Russia; [email protected] (N.K.C.); [email protected] (A.G.V.) 3 Institute for Biological Problems of Cryolithozone, Siberian Division, Russian Academy of Science, 41 Lenina Street, Yakutsk 677000, Russia; [email protected] * Correspondence: [email protected]; Tel.: +7-9021-600-627 Received: 26 May 2020; Accepted: 14 June 2020; Published: 16 June 2020 Abstract: Golden root (Rhodiola rosea L., Crassulaceae) is a famous medical plant with a one-sided history of scientific interest in the roots and rhizomes as sources of bioactive compounds, unlike the herb, which has not been studied extensively. To address this deficiency, we used high-performance liquid chromatography with diode array and electrospray triple quadrupole mass detection for comparative qualitative and quantitative analysis of the metabolic profiles of Rhodiola rosea organs before and after gastrointestinal digestion in simulated conditions together with various biochemical assays to determine antioxidant properties of the extracts and selected compounds. R. rosea organs showed 146 compounds, including galloyl O-glucosides, catechins, procyanidins, simple phenolics, phenethyl alcohol derivatives, (hydroxy)cinnamates, hydroxynitrile glucosides, monoterpene O-glucosides, and flavonol O-glycosides, most of them for the first time in the species. -
Opportunities and Pharmacotherapeutic Perspectives
biomolecules Review Anticoronavirus and Immunomodulatory Phenolic Compounds: Opportunities and Pharmacotherapeutic Perspectives Naiara Naiana Dejani 1 , Hatem A. Elshabrawy 2 , Carlos da Silva Maia Bezerra Filho 3,4 and Damião Pergentino de Sousa 3,4,* 1 Department of Physiology and Pathology, Federal University of Paraíba, João Pessoa 58051-900, Brazil; [email protected] 2 Department of Molecular and Cellular Biology, College of Osteopathic Medicine, Sam Houston State University, Conroe, TX 77304, USA; [email protected] 3 Department of Pharmaceutical Sciences, Federal University of Paraíba, João Pessoa 58051-900, Brazil; [email protected] 4 Postgraduate Program in Bioactive Natural and Synthetic Products, Federal University of Paraíba, João Pessoa 58051-900, Brazil * Correspondence: [email protected]; Tel.: +55-83-3216-7347 Abstract: In 2019, COVID-19 emerged as a severe respiratory disease that is caused by the novel coronavirus, Severe Acute Respiratory Syndrome Coronavirus-2 (SARS-CoV-2). The disease has been associated with high mortality rate, especially in patients with comorbidities such as diabetes, cardiovascular and kidney diseases. This could be attributed to dysregulated immune responses and severe systemic inflammation in COVID-19 patients. The use of effective antiviral drugs against SARS-CoV-2 and modulation of the immune responses could be a potential therapeutic strategy for Citation: Dejani, N.N.; Elshabrawy, COVID-19. Studies have shown that natural phenolic compounds have several pharmacological H.A.; Bezerra Filho, C.d.S.M.; properties, including anticoronavirus and immunomodulatory activities. Therefore, this review de Sousa, D.P. Anticoronavirus and discusses the dual action of these natural products from the perspective of applicability at COVID-19. -
Metabolomics Reveals the Molecular Mechanisms of Copper Induced
Article Cite This: Environ. Sci. Technol. 2018, 52, 7092−7100 pubs.acs.org/est Metabolomics Reveals the Molecular Mechanisms of Copper Induced Cucumber Leaf (Cucumis sativus) Senescence † ‡ § ∥ ∥ ∥ Lijuan Zhao, Yuxiong Huang, , Kelly Paglia, Arpana Vaniya, Benjamin Wancewicz, ‡ § and Arturo A. Keller*, , † Key Laboratory of Pollution Control and Resource Reuse, School of Environment, Nanjing University, Nanjing, Jiangsu 210023, China ‡ Bren School of Environmental Science & Management, University of California, Santa Barbara, California 93106-5131, United States § University of California, Center for Environmental Implications of Nanotechnology, Santa Barbara, California 93106, United States ∥ UC Davis Genome Center-Metabolomics, University of California Davis, 451 Health Sciences Drive, Davis, California 95616, United States *S Supporting Information ABSTRACT: Excess copper may disturb plant photosynthesis and induce leaf senescence. The underlying toxicity mechanism is not well understood. Here, 3-week-old cucumber plants were foliar exposed to different copper concentrations (10, 100, and 500 mg/L) for a final dose of 0.21, 2.1, and 10 mg/plant, using CuSO4 as the Cu ion source for 7 days, three times per day. Metabolomics quantified 149 primary and 79 secondary metabolites. A number of intermediates of the tricarboxylic acid (TCA) cycle were significantly down-regulated 1.4−2.4 fold, indicating a perturbed carbohy- drate metabolism. Ascorbate and aldarate metabolism and shikimate- phenylpropanoid biosynthesis (antioxidant and defense related pathways) were perturbed by excess copper. These metabolic responses occur even at the lowest copper dose considered although no phenotype changes were observed at this dose. High copper dose resulted in a 2-fold increase in phytol, a degradation product of chlorophyll. -
Nitrate Prodrugs Able to Release Nitric Oxide in a Controlled and Selective
Europäisches Patentamt *EP001336602A1* (19) European Patent Office Office européen des brevets (11) EP 1 336 602 A1 (12) EUROPEAN PATENT APPLICATION (43) Date of publication: (51) Int Cl.7: C07C 205/00, A61K 31/00 20.08.2003 Bulletin 2003/34 (21) Application number: 02425075.5 (22) Date of filing: 13.02.2002 (84) Designated Contracting States: (71) Applicant: Scaramuzzino, Giovanni AT BE CH CY DE DK ES FI FR GB GR IE IT LI LU 20052 Monza (Milano) (IT) MC NL PT SE TR Designated Extension States: (72) Inventor: Scaramuzzino, Giovanni AL LT LV MK RO SI 20052 Monza (Milano) (IT) (54) Nitrate prodrugs able to release nitric oxide in a controlled and selective way and their use for prevention and treatment of inflammatory, ischemic and proliferative diseases (57) New pharmaceutical compounds of general effects and for this reason they are useful for the prep- formula (I): F-(X)q where q is an integer from 1 to 5, pref- aration of medicines for prevention and treatment of in- erably 1; -F is chosen among drugs described in the text, flammatory, ischemic, degenerative and proliferative -X is chosen among 4 groups -M, -T, -V and -Y as de- diseases of musculoskeletal, tegumental, respiratory, scribed in the text. gastrointestinal, genito-urinary and central nervous sys- The compounds of general formula (I) are nitrate tems. prodrugs which can release nitric oxide in vivo in a con- trolled and selective way and without hypotensive side EP 1 336 602 A1 Printed by Jouve, 75001 PARIS (FR) EP 1 336 602 A1 Description [0001] The present invention relates to new nitrate prodrugs which can release nitric oxide in vivo in a controlled and selective way and without the side effects typical of nitrate vasodilators drugs. -
Targeting the Deubiquitinase STAMBP Inhibits NALP7 Inflammasome
ARTICLE Received 19 Jul 2016 | Accepted 8 Mar 2017 | Published 11 May 2017 DOI: 10.1038/ncomms15203 OPEN Targeting the deubiquitinase STAMBP inhibits NALP7 inflammasome activity Joseph S. Bednash1, Nathaniel Weathington1, James Londino1, Mauricio Rojas1, Dexter L. Gulick1, Robert Fort1, SeungHye Han1, Alison C. McKelvey1, Bill B. Chen1 & Rama K. Mallampalli1,2,3 Inflammasomes regulate innate immune responses by facilitating maturation of inflammatory cytokines, interleukin (IL)-1b and IL-18. NACHT, LRR and PYD domains-containing protein 7 (NALP7) is one inflammasome constituent, but little is known about its cellular handling. Here we show a mechanism for NALP7 protein stabilization and activation of the inflammasome by Toll-like receptor (TLR) agonism with bacterial lipopolysaccharide (LPS) and the synthetic acylated lipopeptide Pam3CSK4. NALP7 is constitutively ubiquitinated and recruited to the endolysosome for degradation. With TLR ligation, the deubiquitinase enzyme, STAM-binding protein (STAMBP) impedes NALP7 trafficking to lysosomes to increase NALP7 abundance. STAMBP deubiquitinates NALP7 and STAMBP knockdown abrogates LPS or Pam3CSK4-induced increases in NALP7 protein. A small-molecule inhibitor of STAMBP deubiquitinase activity, BC-1471, decreases NALP7 protein levels and suppresses IL-1b release after TLR agonism. These findings describe a unique pathway of inflammasome regulation with the identification of STAMBP as a potential therapeutic target to reduce pro-inflammatory stress. 1 Department of Medicine, Acute Lung Injury Center of Excellence, University of Pittsburgh, UPMC Montefiore, NW 628, Pittsburgh, Pennsylvania 15213, USA. 2 Departments of Cell Biology and Physiology and Bioengineering, University of Pittsburgh, Pittsburgh, Pennsylvania 15213, USA. 3 Medical Specialty Service Line, Veterans Affairs Pittsburgh Healthcare System, Pittsburgh, Pennsylvania 15240, USA. -
The Use of Plants in the Traditional Management of Diabetes in Nigeria: Pharmacological and Toxicological Considerations
Journal of Ethnopharmacology 155 (2014) 857–924 Contents lists available at ScienceDirect Journal of Ethnopharmacology journal homepage: www.elsevier.com/locate/jep Review The use of plants in the traditional management of diabetes in Nigeria: Pharmacological and toxicological considerations Udoamaka F. Ezuruike n, Jose M. Prieto 1 Center for Pharmacognosy and Phytotherapy, Department of Pharmaceutical and Biological Chemistry, School of Pharmacy, University College London, 29-39 Brunswick Square, WC1N 1AX London, United Kingdom article info abstract Article history: Ethnopharmacological relevance: The prevalence of diabetes is on a steady increase worldwide and it is Received 15 November 2013 now identified as one of the main threats to human health in the 21st century. In Nigeria, the use of Received in revised form herbal medicine alone or alongside prescription drugs for its management is quite common. We hereby 26 May 2014 carry out a review of medicinal plants traditionally used for diabetes management in Nigeria. Based on Accepted 26 May 2014 the available evidence on the species' pharmacology and safety, we highlight ways in which their Available online 12 June 2014 therapeutic potential can be properly harnessed for possible integration into the country's healthcare Keywords: system. Diabetes Materials and methods: Ethnobotanical information was obtained from a literature search of electronic Nigeria databases such as Google Scholar, Pubmed and Scopus up to 2013 for publications on medicinal plants Ethnopharmacology used in diabetes management, in which the place of use and/or sample collection was identified as Herb–drug interactions Nigeria. ‘Diabetes’ and ‘Nigeria’ were used as keywords for the primary searches; and then ‘Plant name – WHO Traditional Medicine Strategy accepted or synonyms’, ‘Constituents’, ‘Drug interaction’ and/or ‘Toxicity’ for the secondary searches. -
Rosemary)-Derived Ingredients As Used in Cosmetics
Safety Assessment of Rosmarinus Officinalis (Rosemary)-Derived Ingredients as Used in Cosmetics Status: Tentative Amended Report for Public Comment Release Date: March 28, 2014 Panel Meeting Date: June 9-10, 2014 All interested persons are provided 60 days from the above release date to comment on this safety assessment and to identify additional published data that should be included or provide unpublished data which can be made public and included. Information may be submitted without identifying the source or the trade name of the cosmetic product containing the ingredient. All unpublished data submitted to CIR will be discussed in open meetings, will be available at the CIR office for review by any interested party and may be cited in a peer-reviewed scientific journal. Please submit data, comments, or requests to the CIR Director, Dr. Lillian J. Gill. The 2014 Cosmetic Ingredient Review Expert Panel members are: Chairman, Wilma F. Bergfeld, M.D., F.A.C.P.; Donald V. Belsito, M.D.; Ronald A. Hill, Ph.D.; Curtis D. Klaassen, Ph.D.; Daniel C. Liebler, Ph.D.; James G. Marks, Jr., M.D.; Ronald C. Shank, Ph.D.; Thomas J. Slaga, Ph.D.; and Paul W. Snyder, D.V.M., Ph.D. The CIR Director is Lillian J. Gill, D.P.A. This safety assessment was prepared by Monice M. Fiume, Assistant Director/Senior Scientific Analyst. © Cosmetic Ingredient Review 1620 L Street, NW, Suite 1200♢ Washington, DC 20036 ♢ ph 202.331.0651 ♢ fax 202.331.0088 ♢ [email protected] TABLE OF CONTENTS Abstract ...................................................................................................................................................................................................................................... -
PRODUCT INFORMATION Arbutin Item No
PRODUCT INFORMATION Arbutin Item No. 26407 CAS Registry No.: 497-76-7 Formal Name: 4-hydroxyphenyl β-D-glucopyranoside OH Synonyms: β-Arbutin, NSC 4036 MF: C H O OH 12 16 7 HO FW: 272.3 O Purity: ≥98% UV/Vis.: λmax: 221, 283 nm OH O Supplied as: A crystalline solid Storage: -20°C OH Stability: ≥2 years Information represents the product specifications. Batch specific analytical results are provided on each certificate of analysis. Laboratory Procedures Arbutin is supplied as a crystalline solid. A stock solution may be made by dissolving the arbutin in the solvent of choice. Arbutin is soluble in organic solvents such as ethanol, DMSO, and dimethyl formamide, which should be purged with an inert gas. The solubility of arbutin in these solvents is approximately 1, 10, and 20 mg/ml, respectively. Further dilutions of the stock solution into aqueous buffers or isotonic saline should be made prior to performing biological experiments. Ensure that the residual amount of organic solvent is insignificant, since organic solvents may have physiological effects at low concentrations. Organic solvent-free aqueous solutions of arbutin can be prepared by directly dissolving the crystalline solid in aqueous buffers. The solubility of arbutin in PBS, pH 7.2, is approximately 3 mg/ml. We do not recommend storing the aqueous solution for more than one day. Description Arbutin is a glycosylated hydroquinone that has been found in Arctostaphylos plants and has diverse biological activities, including tyrosinase inhibitory, antioxidant, and anti-inflammatory properties.1,2 It inhibits human tyrosinase activity in crude tyrosinase solution isolated from human melanocytes (IC50s = 5.7 and 18.9 mM using L-tyrosine and L-DOPA as substrates, respectively) as well as in intact 3 melanocytes (IC50 = 0.5 mM). -
Graphical Abstract CG 18-1-MS
Send Orders for Reprints to [email protected] 3 Current Genomics, 2017, 18, 3-26 REVIEW ARTICLE ISSN: 1389-2029 eISSN: 1875-5488 Impact Factor: 2.43 Established Human Cell Lines as Models to Study Anti-leukemic Effects of Flavonoids BENTHAM SCIENCE Katrin Sak* and Hele Everaus Department of Hematology and Oncology, University of Tartu, Tartu, Estonia Abstract: Despite the extensive work on pathological mechanisms and some recent advances in the treatment of different hematological malignancies, leukemia continues to present a significant challenge being frequently considered as incurable disease. Therefore, the development of novel therapeutic agents with high efficacy and low toxicity is urgently needed to improve the overall survival rate of pa- A R T I C L E H I S T O R Y tients. In this comprehensive review article, the current knowledge about the anticancer activities of Received: May 11, 2015 flavonoids as plant secondary polyphenolic metabolites in the most commonly used human established Revised: November 20, 2015 Accepted: November 27, 2015 leukemia cell lines (HL-60, NB4, KG1a, U937, THP-1, K562, Jurkat, CCRF- CEM, MOLT-3, and MOLT-4) is compiled, revealing clear anti-proliferative, pro-apoptotic, cell cycle arresting, and differ- DOI: 10.2174/138920291766616080316 entiation inducing effects for certain compounds. Considering the low toxicity of these substances in 5447 normal blood cells, the presented data show a great potential of flavonoids to be developed into novel anti-leukemia agents applicable also in the malignant cells resistant to the current conventional che- motherapeutic drugs. Keywords: Antiproliferation, Apoptosis, Cell cycle arrest, Cytotoxicity, Differentiation, Flavonoids, Leukemia, Human cell lines. -
Ultrasound-Assisted Extraction Optimization Using
a ISSN 0101-2061 (Print) Food Science and Technology ISSN 1678-457X (Online) DOI: https://doi.org/10.1590/fst.13421 Berberis crataegina DC. as a novel natural food colorant source: ultrasound-assisted extraction optimization using response surface methodology and thermal stability studies Mehmet DEMIRCI1,2 , Merve TOMAS1 , Zeynep Hazal TEKIN-ÇAKMAK2 , Salih KARASU2* Abstract This study aimed to investigate the potential use of anthocyanin of Berberis crataegina DC. as a natural food coloring agent in the food industry. For this aim, the ultrasound-assisted extraction (UAE) method was performed to extract anthocyanin of Berberis crataegina DC. The effect of ultrasound power 1(X : 20-100%), extraction temperature (X2: 20-60 °C), and time (X3: 10-20 min) on TPC and TAC of Berberis crataegina DC. extracts were examined and optimized by applying the Box–Behnken experimental design (BBD) with the response surface methodology (RSM). The influence of three independent variables and their combinatorial interactions on TPC and TAC were investigated by the quadratic models (R2: 0.9638&0.9892 and adj R2:0.9171&0.9654, respectively). The optimum conditions were determined as the amplitude level of 98%, the temperature of 57.41 °C, and extraction time of 13.86 min. The main anthocyanin compounds were identified, namely, Delphinidin-3-O- galactoside, Cyanidin-3-O-glucoside, Cyanidin-3-O-rutinoside, Petunidin-3-O-glucoside, Pelargonidin-3-O-glucoside, and Peonidin-3-O-glucoside. The anthocyanin degradation showed first-order kinetic, degradation rate constant (k), the half-life values (t1/2), and loss (%) were significantly affected by different temperatures (P < 0.05). -
4Nbutylresorcinol, a Highly Effective Tyrosinase Inhibitor for the Topical
DOI: 10.1111/jdv.12051 JEADV ORIGINAL ARTICLE 4-n-butylresorcinol, a highly effective tyrosinase inhibitor for the topical treatment of hyperpigmentation L. Kolbe,* T. Mann, W. Gerwat, J. Batzer, S. Ahlheit, C. Scherner, H. Wenck, F. Sta¨ b Research and Development, Beiersdorf AG, Hamburg, Germany *Correspondence: L. Kolbe, E-mail: [email protected] Abstract Background Hyperpigmentary disorders like melasma, actinic and senile lentigines are a major cosmetic concern. Therefore, many topical products are available, containing various active ingredients aiming to reduce melanin production and distribution. The most prominent target for inhibitors of hyperpigmentation is tyrosinase, the key regulator of melanin production. Many inhibitors of tyrosinase are described in the literature; however, most of them lack clinical efficacy. Methods We were interested in evaluating the inhibition of skin pigmentation by well-known compounds with skin- whitening activity like hydroquinone, arbutin, kojic acid and 4-n-butylresorcinol. We compared the inhibition of human tyrosinase activity in a biochemical assay as well as inhibition of melanin production in MelanoDermÔ skin model culture. For some compounds, the in vivo efficacy was tested in clinical studies. Results Arbutin and hydroquinone only weakly inhibit human tyrosinase with a half maximal inhibitory concentration (IC50) in the millimolar range. Kojic acid is 10 times more potent with an IC50 of approximately 500 lmol ⁄ L. However, by far the most potent inhibitor of human tyrosinase is 4-n-butylresorcinol with an IC50 of 21 lmol ⁄ L. In artificial skin models, arbutin was least active with an IC50 for inhibition of melanin production > 5000 lmol ⁄ L. Kojic acid inhibited with an IC50 > 400 lmol ⁄ L.